Chapter 23: Soils
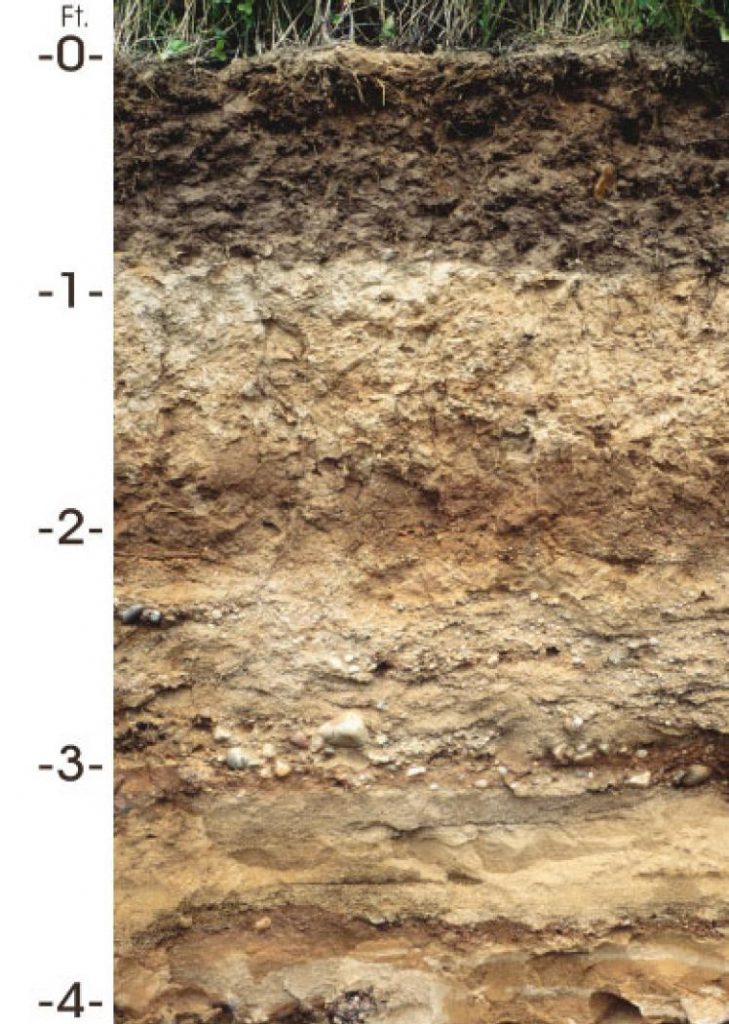
If people had a longer life and were more observant of what is below their feet perhaps they would appreciate that soils (Fig. 1) are living things and that they demonstrate many of the properties that we associate with organisms. They are dynamic entities that develop through time in an orderly and predictable way and are characterized by a number of processes that involve matter and energy transformations. However, they do not have boundaries in space or time and, since they are not discrete, they do not reproduce. In an ecological sense, they are communities possessing a variety of living components that collectively, in conjunction with physical processes, carry out a variety of functions. Observers often consider soils to be an abiotic medium in which organisms grow, but the actions of the organisms in the soil are often so intertwined with the physical processes occurring there that it is pointless to try to separate them, other than to acknowledge that the relative importance of the activities of organisms in the soil vs. the physical processes varies greatly between different types of soils.
Although most consider soils to be an assemblage of debris (i.e., ‘dirt’), soils are better understood if viewed as being composed of the four basic elements that the Greeks and other cultures described: earth, air, water and fire. The earth is a solid material, derived both from inorganic sources, ultimately rock, and from organic sources, pieces of material that are derived from living things. Water pervades the soil as its adhesive properties assure that it sticks to all the particles. Depending on the amount of water, air permeates the soil to a greater or lesser extent. And finally, the fire of the soil is the living creatures, mostly small, highly dominated by fungi and bacteria, but also including a variety of invertebrates, protists, occasional vertebrates and a dynamic population of plant roots. All four of the soils parts are critically important to plants: the solid material is the ultimate source of most nutrients and provides surfaces where significant events occur; water is needed by all plants and also is the medium from which they obtain nutrients; air provides the oxygen that plants and many other living things in the soil require; the living things in the soil carry out a variety of processes significant to plants, in particular, the degradation of large molecules into units that can dissolve in the solution and be available to plants. Each of these four components, earth, water, air and fire, interacts with the others: living things change the gas composition, the gas composition affects living things; water moves and organizes the solid phase; the solid phase controls the water content; the solid components change water chemistry; changes in water chemistry can add or subtract to the solid phase. Soils are a web of interactions, and while components can be listed, the whole is much more than the sum of the pieces and the functions of soil are hard to attribute to specific components.
The D’s of Dirt—Deeds, Dynamic, Diverse
Biologists (and non-biologists!) should be aware of three key aspects of soils: what they do—their deeds; how they are dynamic, and how diverse they are.
TOPICS
- Deeds of the soil — what soils can do
- Dynamics of the soil — how and why soils change
- Diversity of soils
Deeds
What do soils do? A great deal more than we can enumerate here but some of the most important to organisms, populations and communities are: holding water, holding nutrients, and changing the chemistry of the water in the soil.
Soils hold water, usually very significant quantities of water, and this vastly enhances the possibilities for life outside oceans, lakes and rivers. While some terrestrial life exists in areas with no soil (i.e., on bare rock) , their activities are strongly modulated by the availability of water, and water is only available when it is raining and for a short period thereafter. Unless rain is frequent, the activity of living things is very limited because organisms require water and when it is not available they must shut down, becoming inactive and thereby able to tolerate the dry conditions. In contrast, a soil holds and stores water, greatly prolonging the time that water is available to living things and making possible a vast diversity of lifestyles and organisms that would not be present otherwise. Because the soil can absorb and store water, soils moderate the pulses of water flowing overland when precipitation occurs, diminishing the erosion and flooding that would occur without a mantle of soil. This fact is very apparent if one is in a large paved parking lot when rain is falling, or on a parcel of land once the soil has been filled to capacity and can no longer absorb water, or in a situation when rain is falling faster than the soils can absorb it.
The amount of water held by a soil is greatly influenced by the size of the particles that make up the soil. A term that describes the sizes of particles in the soil is texture. Most soils are composed of particles with a variety of sizes, but soils made up mostly of very small particles are called clay soils, and soils composed primarily of larger particles are called sandy soils. There are two main reasons why texture affects the amount of water held by a soil. The first is obvious but often not that significant: texture affects pore space: the total volume between the solid particles which is where water can reside. Obviously, a soil with more pore space has more volume available for water. Surprisingly, the total pore space of different textured soils is not all that different: sandy soils have about the same total pore space as clay soils. What is different between clay soils and sandy soils is the size of the spaces, and this turns out to be particularly important. Clay soils have many small spaces where water can reside while sandy soils have fewer spaces for water but they are considerably larger.
Consider two soils that occupy the same volume, both with ‘no‘ water (although it turns out to be impossible to get rid of all the water). The soil can be filled with water so that all the pore space (spaces between the solid particles) are occupied with water. A soil at this state is said to be saturated (Fig. 2) and one could determine the total pore space by keeping track of how much water had to be added to the soil to saturate it. A soil can only be saturated if it is in a closed container, one that gravity cannot pull water out of. If holes are opened in the bottom of the container then the force of gravity can pull out water. Significantly, gravity cannot pull out all of the water, but it can pull out some of it. It turns out that gravity can remove much more water from the sandy soil than from the clay soil. This is because water is held in small pores much more tightly than water held in large pores, and the force of gravity is only strong enough to pull water from the largest pores. A soil holding all the water that it can against the force of gravity is said to be at field capacity (Fig. 3). To remove more water from the soil one needs to add plants. One could also wait for evaporation to remove more water but this is generally much slower than allowing plants to do it and allows one to see another critical point in soil moisture. Plants, like gravity, can only remove some, but not all, of the water remaining in the soil. This is because eventually the soil becomes so dry that plants cannot survive. Soil at this degree of dryness is said to be at a permanent wilting point (Fig.4). Plants do differ in how much dryness that they can tolerate but most plants, in particular crop species, have quite similar tolerances. Both field capacity and permanent wilting point define degrees of dryness in the soil and actually can be defined in terms that relate to the force holding water in the soil and to a thermodynamic term defined as water potential. And the amount of force present is related to the size of pores that still hold water: as soil dries the water remaining in the soil is in smaller and smaller pores and is harder and harder to remove. Knowing how much water is in a soil is not particularly useful: a clay soil with 15 grams of water per 100 grams of soil (percent moisture = 15%) is so dry that few plants could live in it. A sandy soil with a water content of 15% may be saturated and gravity could remove water from it. Consequently, the ‘wetness’ of a soil is monitored not by water content (percent moisture) but in energetic (water potential) terms.
- Fig. 2 This figure represents a saturated soil: all the spaces between the soil particles are filled with water.
- Fig. 4 Plants growing in soil can remove water from a soil at field capacity. They can exert a pull strong enough to remove water from ‘medium-sized’ pore spaces. But eventually, the only water that remains is in small pores and most plants cannot pull hard enough (see Chapter 24)to remove this water and they die (aka ‘permanently wilt’) at a certain level of soil dryness.
- Fig. 3 If the soil is able to drain gravity will remove water from a saturated soil and the water that remains will be present surrounding the soil particles, because of adhesion, and also in spaces between soil particles, because of cohesion water sticks to itself. The amount of water that gravity can remove and consequently the water ‘held’ by the soil, depends on the size of the spaces, the pores, between the soil particles. Gravity can remove water from the big pores just as gravity will pull water out of a straw. But gravity cannot remove water molecules out of small pores because the cohesive forces of water exceed the force of gravity.
The amount of water held by a soil between its field capacity and the permanent wilting point is important because it represents the storage capacity of the soil that is useful to plants. Water added to a soil that is a field capacity will drain out of the soil due to gravity (how quickly this happens depends on the texture of the soil). Water held in the soil below the permanent wilting point is unavailable to (most) plants. Sandy soils dry out quickly because they store little water between field capacity and permanent wilting point. Clay soils can hold much more but because water moves slowly through the clay soils are generally not desirable for agriculture—the best agricultural soils are described as loams, with a mixture of sand and clay.
In addition to water, soils also hold nutrients. Remember that all the nutrients that plants acquire, with the exception of carbon, come from the soil solution. Thus the water held by the soil represents not only a supply of water but also a supply of nutrients. Exactly how much of each nutrient (and other solutes) are present depends partly upon the amount of water but also on chemical interactions in the soil. A simple view of soil chemistry is that nutrients can be in one of two situations: solids (i.e., part of the soil particles) or solutes, dissolved in the water. There are a variety of mineral salts that can disassociate, putting ions in the soil solution, e.g., Na+ and Cl–. The reality is more complex. The soil is a three-phase system with chemicals not just in the solid-state (precipitated state) and in solution as dissolved ions. A third phase, in between these two, is described as an ‘ion exchange surface’ that is the result of solid components of the soil breaking down (weathering) and losing (generally) cations, producing a negatively charged surface that can electrostatically bind cations, forming a ‘cation exchange surface’. The movement of ions from the soil solution onto this surface is less specific and more dynamic than the precipitation of ions from the soil solution into specific minerals. While precipitation to a specific minerals requires a match between cation and anion, any positively charged ion (cation) can associate with a cation exchange surface. Which cations are actually held depends on their abundance in the soil solution, their size, and the amount of charge. For plant nutrition, the key parameter is abundance in the soil solution. Consider a soil solution in equilibrium with a cation exchange surface and consequently having a certain ratio of Na+ to K+ in the soil solution. If plant roots remove K+ from the soil solution, the lowering of the K+ concentration in the soil solution increases the Na+ /K+ ratio and causes Na+ ions to be exchanged for K+ ions on the cation exchange sites. This replenishes the supply of K+ in the soil solution. A common application of cation exchange surfaces is in water softeners, devices that remove the calcium and magnesium from water and replace them with sodium, thereby making the water ‘softer ’. This results in a number of favorable consequences, e.g., more effective washing with soaps. A water softener operates by moving water through an ion-exchange ‘column’ that has been ‘loaded’ with Na+ (i.e., all the ion exchange sites are filled with Na+). As the water moves through the column the Na+ replaces the Ca2+ and Mg2+ in the solution. Eventually one needs to replace the ion exchange material because it has become ‘filled’ with calcium and magnesium ions.
Hence there are three ‘pools’ of plant nutrients in the soil: specific solid m aterials, both organic and inorganic, the soil solution, and ion exchange sites. Ion exchange can help buffer changes in nutrient supply and explains why soils with higher cation exchange capacity often are generally better soils for agriculture (i.e., can grow better crops). Most ion exchange surfaces are negatively charged and hence are cation exchange surfaces. The amount of ion exchange surfaces present in a soil is strongly dependent on the age of the soil. As soils age, specific minerals are produced by the weathering of the soil minerals and the decomposition of soil organic material. Remember that the soil as a whole, and the soil solution specifically, remains neutral: positive charges equal negative charges. This is also true of the solution that enters (e.g., rainfall) and exits the soil as groundwater, but the chemistry of the water flowing out of the soil may be quite different from that entering the soil.
Ultimately the supply of nutrients (e.g., K+, Ca2+, PO4–, SO42-) in the soil depends upon the balance between additions and losses. Processes that add nutrients include: additions from rain, snowfall and dust; decomposition of organic material into components that are able to dissolve in the soil solution; weathering of soil minerals into components that are able to dissolve. Processes that remove nutrients from the soil include erosion, leaching (the loss of solutes in water as gravity pulls water out of the root zone), and the harvesting / removal of plant or animal material.
Dynamics of the soil
Soils are continuously changing as a result of a variety of processes. Solid material is continuously being added, primarily from the plants that shed leaves, stems, fruits and entire bodies to the soil surface and continually add roots directly within the soil. Material is also added by animals and by mass processes e.g., wind and water deposition. Some of the material is readily decomposed, disappearing into the atmosphere (carbon dioxide) and soil solution (ammonia, ‘dissolved organic matter’) within a few days. Other materials (e.g., tree trunks, large woody roots) remain for hundreds of years. Water is continually flowing through the soil, usually being deposited on the surface by rain/snow and moving down with the pull of gravity. B ut occasionally water moves upwards because of evaporation from the surface of the soil. As water moves, it carries material with it, mostly in solution but sometimes in suspension (if there is a mass flow). Carried materials are not necessarily transported out of the soil but may be deposited, generally in lower layers, where the physical conditions (amount of oxygen, pH, size and type of particles) may be different. While the water balance of a soil is generally zero (i.e., inputs match outputs) over the course of a year, this is generally not the case for solid material and soils may be either accumulating or losing material. Even if the solid and liquid phases are in a steady-state, with losses matching gains, activities in the soil can change its structure. While we generally think of material in the soil as breaking down because of the processes of weathering and decomposition, sometimes larger molecules are made from smaller ones and existing soil particles may fuse with each other.
Diversity of soils
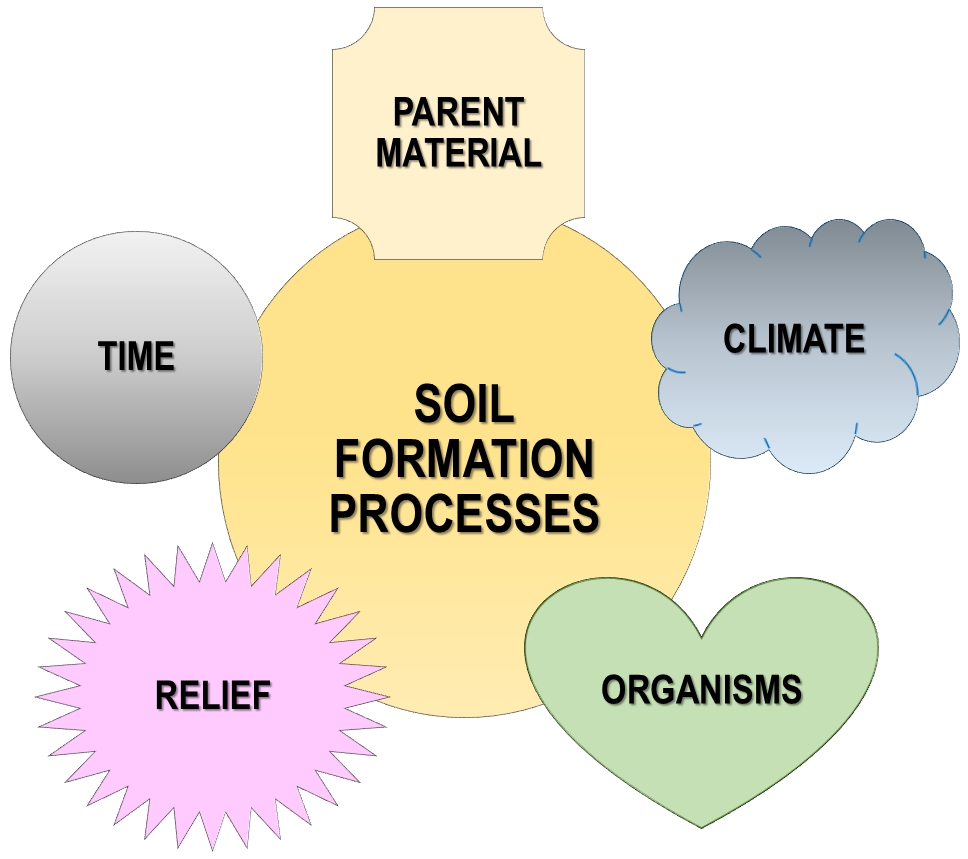
Because soils are dynamic, they are diverse and change through time in predictable ways, i.e., they develop over time. Young soils will have different features than old ones. The age of the soil is one of the five key factors that determine the nature of a soil (Fig. 5). The other four are: parent material (what it is made of), climate, biota, and slope. Parent material can vary between solid rock (e.g., a lava flow), particulate mineral material (e.g., volcanic ash), or organic matter (e.g., in a bog) with a wide variety in between. Parent material affects particle size, soil chemistry and what organisms are likely to occupy the soil. Climate, i.e., patterns of rainfall, temperature and the variation in these factors, is important for reasons that should be apparent: temperature controls the rate of decomposition and weathering; rainfall also influences decomposition and weathering and also controls the amount of water percolating through the soil. Biota, the forms of life present, influences the types of organic material that are deposited and the rates of decomposition. The remaining factor of importance, slope, is perhaps is surprising until one appreciates that all of the following are influenced by it: the amount of water running through the soil, whether or not water may be stagnant on/in a soil, the amount of erosion/deposition on a site.
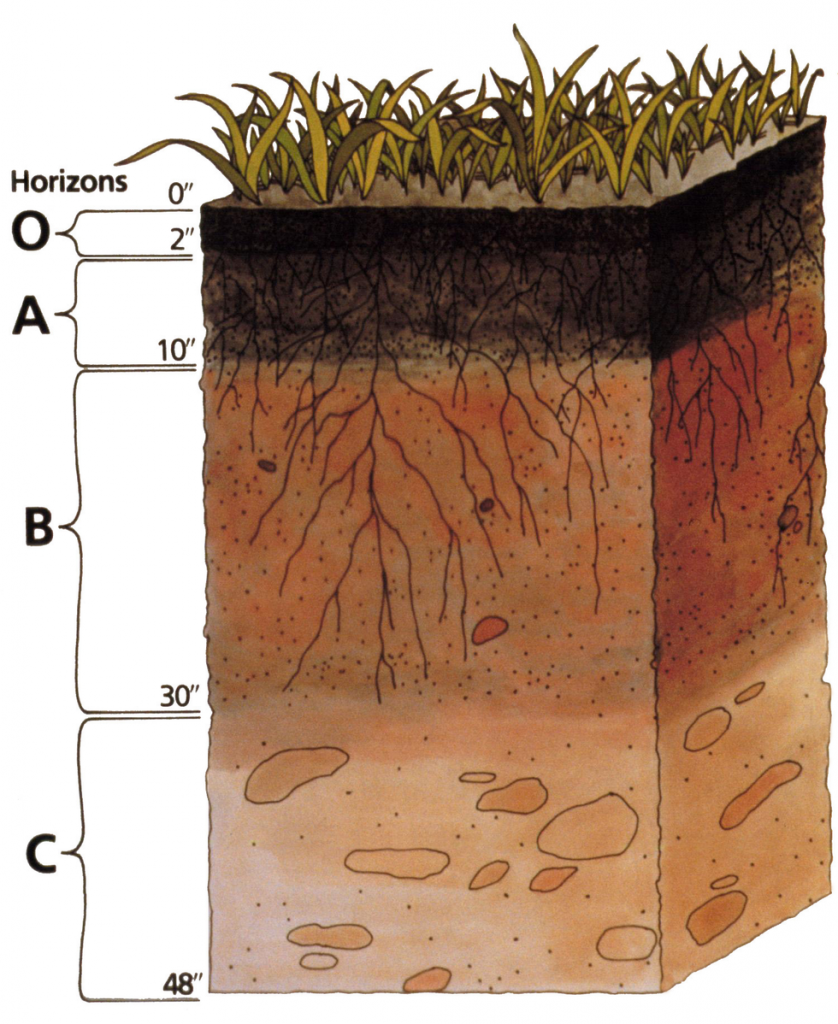
Because of variation in the factors described above, soils are diverse. Moreover, their features are changing continuously depending upon their age. One manifestation of this diversity is the existence of layers (horizons) in many soils. The horizons develop because of processes taking place in the soil.
In many parts of the world, including the north central and northeastern U.S. glaciers played a very significant role by influencing three of these factors: In many areas, they eliminated whatever soil was present, thus many soils are relatively young; the glaciers deposited a variety of soil materials (ranging from sands to clays) on which new soils developed; and glaciers created a variety of topographies (slopes) upon which soils developed.
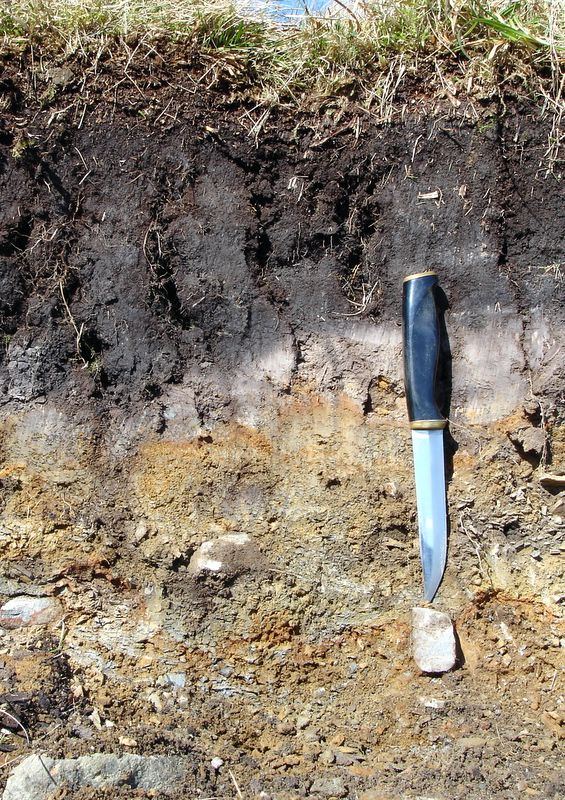
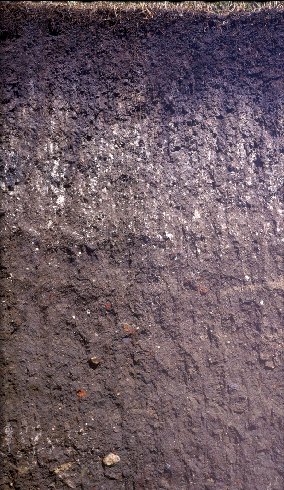
Media Attributions
- Soil (Antigo) © US Gov is licensed under a Public Domain license
- Soil formation factors © Ivtorov is licensed under a CC BY-SA (Attribution ShareAlike) license
- Soil Profile © US Department of Agriculture is licensed under a Public Domain license
- Mollisol © US Department of Agriculture is licensed under a Public Domain license