Chapter 26: Interactions Involving Conditions
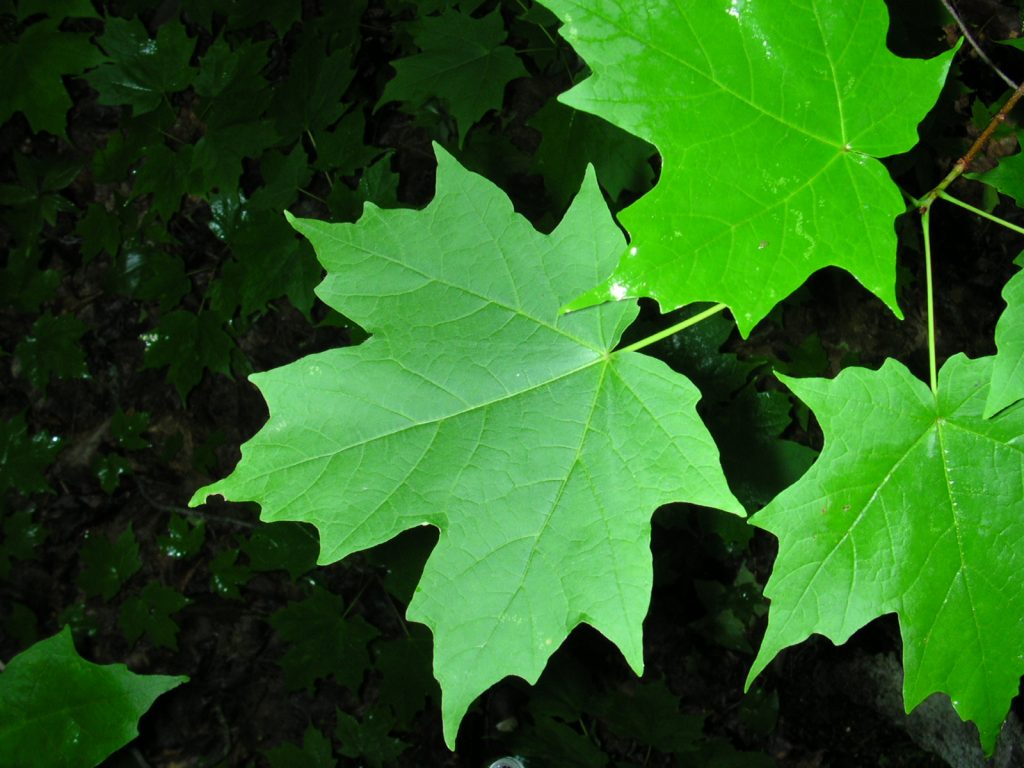
A particularly significant and interesting aspect of organismal life is its interaction with its surrounding environment. As indicated by the term, interaction implies that both components affect each other: the environment affects organisms and organisms affect the environment. The ‘environment’ includes components that are living, i.e., other organisms (the biotic environment), and components that are non-living such as rocks, clouds, water, dead organisms or parts of dead organisms. The environment has properties, or conditions, such as temperature and oxygen concentration that are commonly considered ‘the abiotic environment’ or the ‘physical environment’. Conditions are the consequences of physical processes such as radiation, diffusion, convection; and these processes are sometimes strongly influenced by biotic processes (Fig. 1), such as respiration (generates heat and carbon dioxide, removes oxygen) and photosynthesis (absorbs light, adds oxygen and water, removes carbon dioxide). The conditions present on a site can dictate whether or not an organism can exist and, if it exists, how it behaves. This is sometimes described as an interaction between organisms and the physical, or abiotic, environment.
Because organisms can affect conditions, they can interact with other organisms as a result. In addition to the interaction between organisms mediated through conditions, there are more direct interactions between organisms, such as one organism eating another or an insect transporting pollen from one plant to another. Interactions are fundamental to the discipline of ecology. Indeed one definition of ecology is ‘a study of the interactions between living things and their environment’.
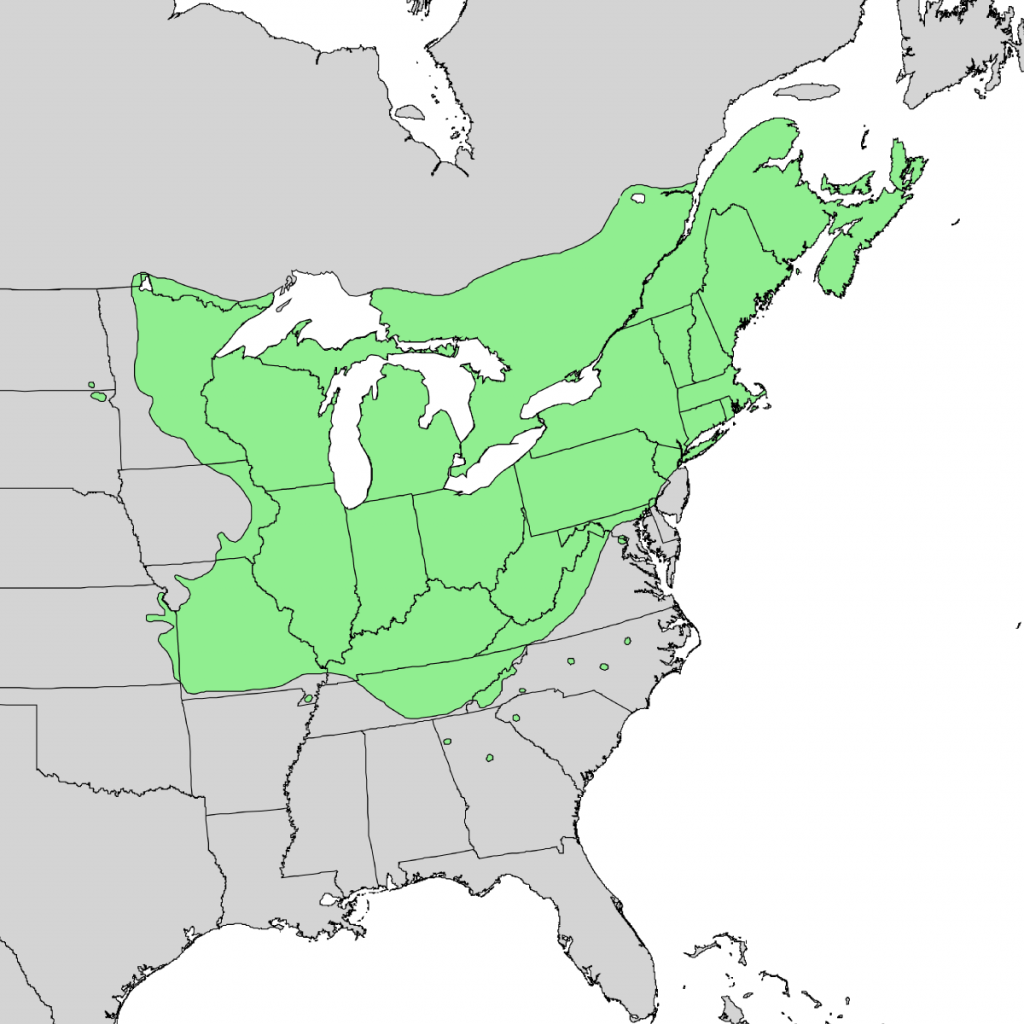
Another common definition of ecology is ‘a study of the distribution and abundance of organisms’ (Fig. 2). The distribution and abundance of organisms are controlled by interactions between organisms and their biotic and abiotic environment. For example, the distribution of a plant might be limited by the intensity of light. The amount of light available is a consequence of both the physical environment (latitude, degree of cloudiness, whether the site is facing south or north, etc.) and the biotic environment (presence or absence of tree species to intercept the light). The distribution and abundance of a particular plant (species ‘A’) might also be limited to the presence or absence of an organism that eats the plant or perhaps by an organism that pollinates the plant. In either of the latter cases, one might consider the factors that determine the distribution and abundance of the second species to be controlling the distribution and abundance of species A.
Another definition of ecology is “a study of biological organization above the level of individual.” As we have seen, organisms have a structure (form, size, organization of component parts), and also organisms have functions, they do things such as develop, reproduce, and exchange matter and energy with their environment. Similarly, groups of organisms (e.g. populations) have structural and functional features that can be described and categorized, and explanations can be sought as to what determines their organization. An important point is that life is not simply organized at the level of the cell and the level of an organism; there also is an organization involving groups of organisms and this is the level of study for the discipline of ecology.
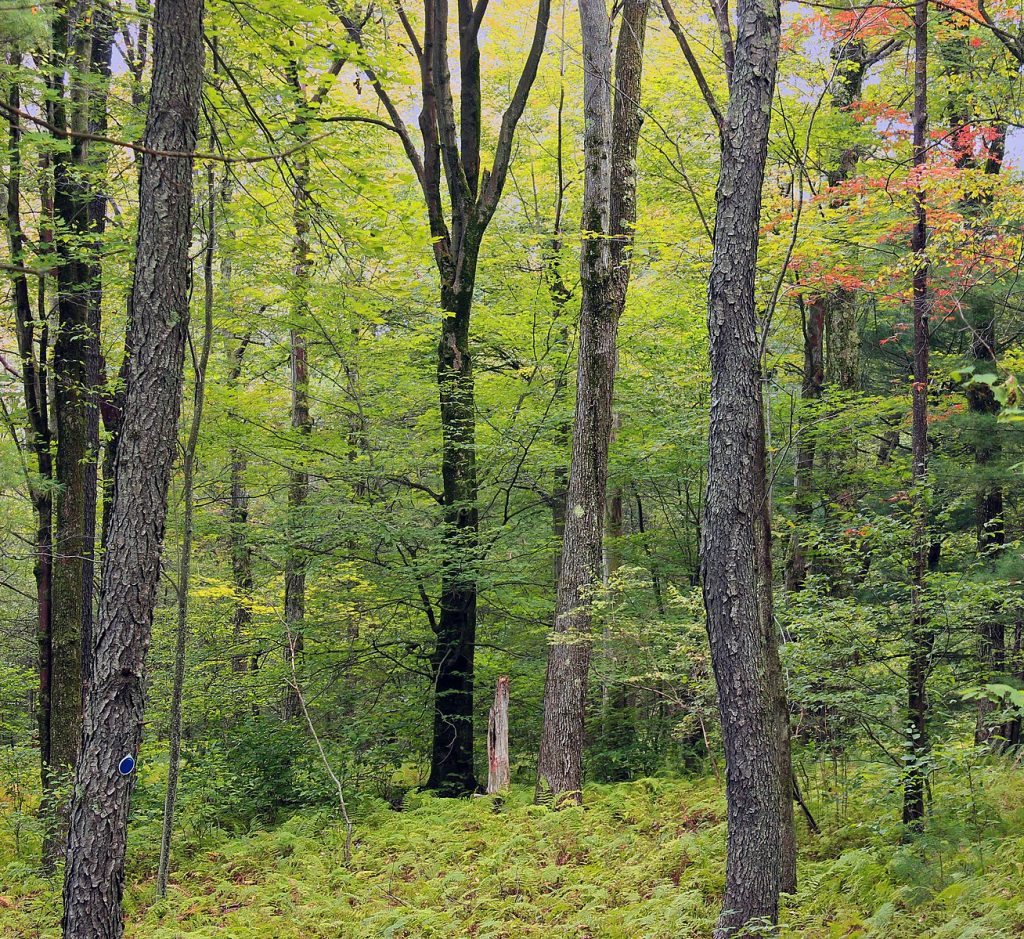
Two entities that ecologists study are populations (groups of individuals of the same species) and communities (assemblages of species in a particular area). A population could certainly be considered a ‘living thing’ and its distribution across the landscape is a manifestation of biological organization and is a structural feature of this entity. The abundance of a species (how many individuals are present per unit of the environment) is another structural feature of a population. The fact that populations have patterns of distribution and abundance represents an organization, a structure, and the processes determining this structure are the same processes discussed above: interactions between organisms and their physical and biotic environments. Similar arguments can be made concerning the organization of communities.
The final chapters of this book examines of the role of interactions in the biology of organisms. This chapter considers the interactions between organisms and conditions, examining the key conditions that affect terrestrial and aquatic habitats, why these conditions affect an organism’s function, and what factors cause these conditions to vary, including how organisms themselves may control conditions. In the next chapter, we will examine more direct interactions between organisms, trying to see fundamental similarities in the ways that organisms interact with each other. Finally, chapters 28-31 examine agriculture, an interaction that we all depend upon, and interactions that are critical to agriculture.
Our approach will be centered primarily on organisms, although many of the topics overlap with ecology and approach the topics from a broader scale.
TOPICS
- Temperature
- Temperature’s influences
- Organism tolerances
- Organism growth
- Psychophiles and thermophiles
- Fruit ripening
- Temperature as a cue
- Temperature’s influence on other conditions
- Moisture
- Terrestrial habitats
- Aquatic habitats
- Salinity
- Oxygen
- Light
- Currents
- Nutrients
Temperature
Temperature is of critical importance to all organisms—it affects whether they can survive and what they do. Even for organisms who regulate their temperature at a set point, (i.e., homeotherms), temperature has significant consequences. But temperature is even more significant for the vast majority of organisms who do not regulate their temperature. For such organisms, termed poikilotherms, the thermal conditions of their environment control their metabolic activity. Although the temperature is important in all habitats, it is of relatively less importance in most aquatic systems because the thermal properties of water buffer temperature fluctuations. But organisms in terrestrial habitats are immersed in a fluid (air) that absorbs very little heat energy and has a low heat capacity (i.e., little buffering ability). This makes the temperature of terrestrial systems much more dynamic in time and space than in most aquatic systems. One of the key reasons why temperature is important in terrestrial habitats is because it affects evaporation and moisture levels. While there are some terrestrial habitats where temperature is not a key determinant of activities, from a global perspective temperature and moisture are the major factors controlling what organisms are present and what they are doing.
Temperature’s Influences on Organisms
All organisms have a range of temperatures in which they can carry out the functions that define and sustain them. Temperatures above and below this range alter their structure and functioning in several ways. The most important alterations are listed below.
Membranes and temperature
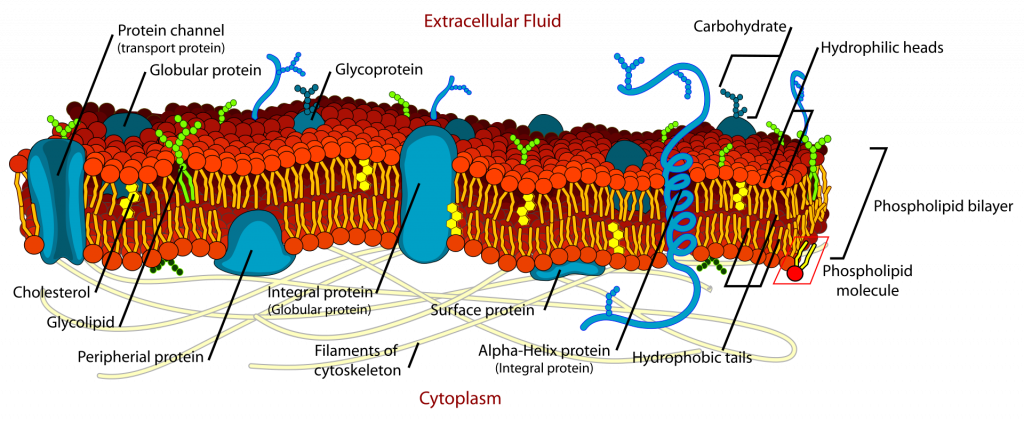
Membranes are essential for life, they regulate molecular movement and perform a variety of other functions. The physical nature of membranes is between a solid and a liquid; they can be described as “ liquid crystals” because part of their nature is rigid and ordered while other parts are fluid-like (Fig. 4, discussed also in Chapter 3). This duality of structure is important to their function; they need to be partly rigid because much of their functioning depends upon the organization of its parts; if these parts are out of place, functioning is disrupted. At the same time, membranes need to be able to change shape and membrane components do need to be able to move laterally; this is only possible because of membrane fluidity. Temperature disrupts the balance between fluidity and rigidity—high temperatures make membranes more fluid and low temperatures make them more rigid (crystalline). Shifts in either direction are damaging. Membrane characteristics of organisms found in habitats of different temperatures are different in predictable ways that relate to the maintenance of a certain degree of ‘fluidity’. In organisms living at higher temperatures, the lipids are more likely to contain longer chains of hydrocarbon and these chains are more likely to be more saturated. Both of these features make the membranes that they are found in more ‘solid-like’ at any particular temperature. Along the same lines, it has been found that some organisms that experience yearly variation in temperature adjust their membrane chemistry in a way that maintains a constant degree of fluidity in spite of changing temperatures. Thus, the range of temperature tolerance is partly determined by the chemical nature of an organism’s membranes.
Coordinated chemical reactions and temperature
A second factor involved in both high and low-temperature disruptions of organism function is the balancing of the myriad chemical reactions that are taking place inside cells. The rate of nearly all chemical reactions is strongly influenced by temperature, with the rates going up as the temperature goes up. Most of these reactions are in some sort of a balance so that, in general, there is no build-up or depletion of metabolites. This balance can be upset at both high and low temperatures because the temperature sensitivity is not the same for all reactions—thus reactions that are ‘in balance’ at some temperatures may not be at higher or lower temperatures. The biochemistry of cells is such that control process (e.g., feedback loops) can operate to achieve balance in metabolic pathways, but there are limits to these control processes, and at least some of the problems with high and low temperatures may be attributed to problems with reactions becoming ‘unbalanced’.
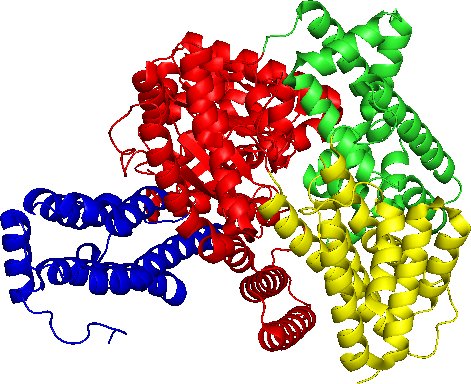
Enzymes and temperature
Enzyme functioning is dependent upon a three-dimensional structure. This structure can be disrupted by high temperatures in a process called denaturing because the thermal motion becomes sufficient to break the relatively weak bonding that accounts for certain aspects of the enzyme structure. The temperature at which denaturing occurs varies with proteins but is in the range where temperatures become lethal to most organisms (25-40 C).
Problems with freezing
Not surprisingly, freezing causes a variety of problems for living things. The expansion associated with freezing can burst cells, destroying membranes and walls in the process. For plants and fungi, which have a substantial volume of their structure (the apoplast) that is outside of the membranes, freezing (especially if it occurs slowly) generally occurs first outside the cytosol, i.e., in the apoplast, because of its substantially lower solute concentration. The freezing outside causes the diffusion of water from the inside to the outside, resulting in desiccation damage on the inside. Because of these effects, a wide group of plants and fungi have their lower thermal limit at 0 C, or slightly below it.
In spite of this, plants and fungi do live in habitats where freezing occurs. For some, survival is the result of an overwintering part that is below-ground where temperatures are more moderate and never go below freezing. For others, e.g., trees and shrubs, the existence of perennial above-ground parts reflects an ability to withstand prolonged periods of sub-freezing temperatures. Tissues/cells may avoid freezing through two mechanisms, one involving freezing point depression and the other involving supercooling. Freezing point depression is one of the four ‘colligative properties of solutions’ — changes in the properties of a solution that occur with the addition of solutes, regardless of what solute is added. Although the presence of solutes in the cytosol does lower the freezing point, the effect is relatively small (up to -2 C at the concentrations of solutes typically found in the cytosol) , and protection against freezing by this mechanism is not significant for most organisms. Supercooling can result in much more substantial drops in the temperature at which freezing occurs. Supercooling describes a situation where liquid water exists at temperatures where it is usually frozen. Although supercooling can occur in pure, or nearly pure, water, especially if there are no sites for ice nucleation (e.g., under certain atmospheric conditions that cause supercooled raindrops), in living systems supercooling appears to be a consequence of specific antifreeze solutes, proteins or glycoproteins, that somehow prevent crystal formation. Such compounds are found not only in plants but also in fish and insects. The lower limit of supercooling is around -40 C (which is also -40 F!!!!), but for many species the limit of supercooling is well above this, in the range of -10 C.
Other problems caused by freezing are particularly significant to vascular plants and relate to water transport. Water cannot flow in the xylem if it is frozen, and, as mentioned above, the lower solute concentration of the apoplast, and particularly the xylem transport cells, means that water transport becomes impossible when the temperature falls below zero. Since water loss to the atmosphere is almost always occurring because the air is drier than the plant, desiccation will result. This probably accounts for a common linkage between freezing tolerance and drought tolerance. An additional problem related to water transport is that when water freezes dissolved air is excluding from the ice, creating bubbles of air in the ice. Upon thawing, these bubbles remain. This is potentially a problem for xylem transport because the tensions that develop during xylem transport will cause expansion of the bubbles and cavitation (air locking) of vessels and tracheids, disrupting xylem water transport. This problem may be avoided by the production of new water-conducting cells in early spring, by a pressurization of the xylem (‘root pressure’) that occurs in at least some species as a result of solute absorption in the spring, or as a result of physical processes that can eliminate the bubbles.
Tolerances
All organisms have high and low thermal limits; if an organism reaches that temperature it dies. Chronic exposure to more moderate temperatures can also be lethal, in a manner connected to the length of exposure. Some plants will be killed by a short-term exposure at 38 C, but 36 C is lethal if exposure is longer than 60 minutes.
Extreme tolerances
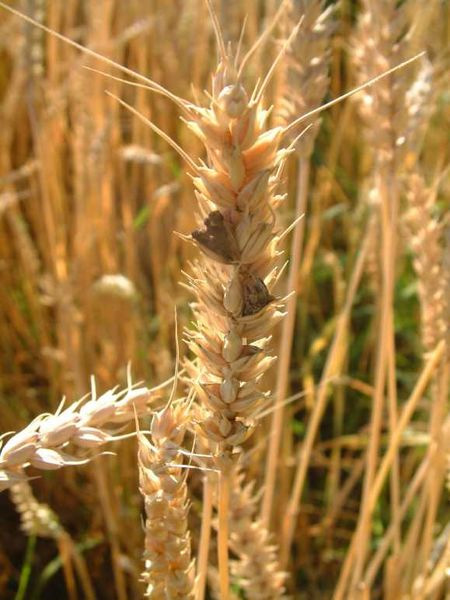
The majority of organisms have high-temperature tolerances of around 40 C and low-temperature tolerances of 0 C but there are stages of many organisms that have a much wider range of tolerance. This tolerance is often associated with a stage in the organism’s life cycle that is devoted to dispersal, e.g., spores, seeds. These structures serve to perpetuate the organism through a time of unfavorable conditions. Along with tolerance to temperature extremes, there typically comes a tolerance to desiccation and to a number of ‘insults’ that would normally kill cells (e.g., ultraviolet radiation, extreme pH, lack of oxygen). This tolerance is a consequence of an altered cellular structure that simultaneously increases tolerance and decreases metabolic activity. In short, the structure of the cell(s) becomes more and more inanimate and less and less affected by extreme conditions. Usually this state of ‘suspended animation’ involves several or all of the following: accumulation of materials such as starch or oils that serve as reserves of energy and reduced carbon, changes in membrane structure, changes in protein structure, changes in organelles (in eukaryotes), reduction in cytoplasmic volume, desiccation, and, for certain cells, a thickening of the cell wall (or sometimes the addition of a wall to a cell that previously lacked one).
Generally, these tolerant structures are single cells but in some organisms tissues or the entire organisms undergo comparable changes in structure and function. In the plant kingdom, such tolerant tissues are usually present in seeds and sometimes present in apical meristems. Such tolerance can occasionally be found in other structures and can also be found in entire plants, especially those found in freezing environments or deserts. The table below lists prokaryote, protist, fungal and plant structures that are particularly tolerant of extreme conditions. Although the table only tabulates low-temperature tolerances, it is important to realize that, in general, high-temperature tolerance, as well as desiccation tolerance, correlates with low-temperature tolerance. For example, most seeds readily tolerate prolonged exposure to frozen conditions; they also tolerate desiccation and exposure to high temperatures (e.g., 50 C) that would normally kill plants.
group |
structure |
notes |
some bacteria | endospores | most are very resistant to high and low temperatures and survive for prolonged periods |
most bacteria | microbial cyst (=exospore) | bacterial cells increase the thickness of the cell wall and contract the volume of the cytoplasm |
cyanobacteria | akinete | an akinete is a specialized spore found only in some cyanobacteria |
euglenoids | cyst | cyst formation is often triggered by changes in nutrient levels |
dinoflagellates | cyst | cysts have no flagella and produce a cell wall with cellulose, features that are NOT normally present in dinoflagellates |
diatoms | resting cells and spores | spores have thickened silica walls, resting cells do not; these structures may have requirements for germination. Spores of marine forms may be important components of the fossil record |
zygomycetes | spores, zygospores | the zygospores that are associated with sex and are multinucleate have a much thicker cell wall and are more tolerant of abuse than normal cells |
basidiomycetes | both asexual and sexual spores | in addition to spores, some forms produce sclerotia, desiccated and modified hyphae that are inactive and tolerant of extremes |
ascomycetes | both asexual and sexual spores | (see above description for basidiomycetes) |
mosses | whole plants, spores | the spores of most species are tolerant of a variety of treatments; in addition, the entire gametophyte plant of many mosses tolerates both freezing temperatures and desiccation |
ferns and other seedless vascular plants | spores, rarely whole plants | unlike mosses and like most seed plants, ferns generally canNOT tolerate desiccation, although spores of ferns tolerate this and other extreme treatments. Relatively few species have above-ground parts that overwinter in sub-freezing conditions. |
conifers | pollen grains, seeds, whole plants | as a group, many conifers are more tolerant of freezing than most flowering plants, evidenced by the presence of conifers at high latitudes and elevations; however, spores are NOT especially tolerant; they generally only occur in hydrated tissues; |
cycads | seeds only for most species | only a few can tolerate temperatures below freezing, most are restricted to warmer areas |
gnetophytes | seeds only | only Ephedra tolerates freezing temperatures |
ginkgo | pollen, seeds, above-ground parts | the tree is tolerant to the USDA’s zone 3, which has temperatures down to -40 |
flowering plants | pollen, seeds, above-ground parts of some species | quite a number of flowering plants have adapted to temperatures well below freezing; there are also a number of species that can tolerate temperatures above 40 C |
Temperature’s Influences on Growth
Because temperature affects the rate of chemical reactions and because chemical reactions determine what an organism does, and in particular the rate at which things are done, temperature has a profound effect on organism functioning. The combined effects of an organism’s chemical activity are termed metabolism and metabolic rates commonly double to triple with a 10 C (18 F) rise in temperature. While most students generally assume that the opportunity to ‘do more’ as a consequence of a higher metabolic rate is a desirable thing, this isn’t always the case. Higher metabolic rates require more food because an organism’s metabolism runs on cellular respiration. To a certain extent, poikilotherms survive periods of low temperatures because it costs very little to maintain them under these conditions. Lower metabolic rates mean lower oxygen needs; consequently, many plants can survive low oxygen conditions (typically brought about by flooding) much more readily if temperatures are low.
Growth and growing degree days For any organism one of its most significant activit ies is growth, the acquisition of materials, and subsequent utilization of matter and energy to make the organism larger and ultimately coupled with the production of new organisms. The effect of temperature on growth is nicely seen in the concept of growing degree days, a statistic that integrates time and temperature and is used to predict the progress of a wide variety of crops during the season. While the details vary between crops, and even between different varieties of a specific crop, the basic idea is that you can predict the growth stage of a crop species by keeping track of the number of days that the crop has spent at different temperatures. For example, if the daily temperatures had a high of 86 F and a low of 70 F, corn might take 100 days to reach maturity (i.e., the time to harvest); if the temperatures were cooler, with a daily high of 80 F and the low of 60 F, it might take 140 days. Agronomists have developed models to predict how long the crop will take to reach maturity based on the accumulation of something called a ‘growing degree day’. Based on its temperature, each day is assigned a certain number of growing degree days (GDD’ s), with warm days earning more than cold ones. A typical formula that calculates growing degree days is the following:
GDD = [(Tmax + Tmin)/2 ] – 50
Given the days listed above, the warmer day is worth (86+70)/2 – 50 = 28 GDD, and the cooler day is worth (80+60) – 50 = 20 days. If corn needs 2800 GDD days to reach maturity it will do so in 2800/28 = 100 days at the warmer temperatures and 2800/20 = 140 days at the slightly cooler temperatures.
This is an example of an ‘empirical model’, one that attempts to predict things based on observations but not necessarily based on an understanding of how a system operates. The fundamental basis for the model is the observation that crops grow faster when it is warmer. The model is successful in spite of the fact that it has a fairly crude approach to the relationship between growth and temperature. There are a number of features of the model that are useful to appreciate:
- a GDD represents a unit of growth and the basic idea is that plant development can be represented as an accumulation of these units of growth.
- a GDD also represents the product of time and temperature; although this is represented in its units (‘degree-days’), it isn’t obvious in the equation above because the time factor is always 1 day and is not included; the equation might be written:
- GDD = [(Tmax + Tmin)/2 -50] degrees * [1.0] day
- the temperature term has two components, the first represents a type of average, a representation of a dynamic (changing with time) variable, in this case, temperature. This particular average (sum the extreme values and divide by 2) is convenient and it is commonly used by meteorologists to reflect daily temperature. Although it is a crude type of average, based only on two values, it works well. The other temperature term (50 in the above equations) might be described as a ‘base temperature’, the minimum temperature at which no growth occurs. Although the equation potentially predicts an impossible ‘negative growth,’ if the average temperature is below the base temperature, this prediction is rarely the case because in most situations crops aren’t planted until after average temperature daily temperature exceeds the base temperature.
- the GDD concept has been used successfully for a wide variety of crops and also with insects, generally with modifications of the ‘base temperature’ and/or changes in the average temperature term (e.g., letting the maximum temperature never exceed 86)
If one tried to devise a ‘mechanistic model’, one that operates based on the mechanisms of growth, it would be much more challenging. Growth is a complex process that is a consequence of a variety of chemical reactions occurring simultaneously. Most of these are clearly affected by temperature but predicting the net effect of temperature on all of them would be particularly challenging. Certainly, the processes associated with growth are controlled by temperature, but the interrelationships associated with growth would be difficult to elucidate.
Variation in the temperature ranges for growth While the vast majority of fungi, plants and protists have an optimum temperature for growth in the range of 25-35 C, there are some exceptions. A number of plants have maximum rates of growth well below 35 C. Not surprisingly, these plants grow in cooler habitats. While there are a number of possible reasons for this, including the possibility that these organisms have membranes that function more appropriately at lower temperatures (see above), or the interaction between temperature and moisture (see below), another explanation involves the temperature sensitivity of photosynthesis and respiration. As mentioned above, respiration is closely tied to temperature and for most plants reaches a maximum in the 30-35 C range. The response of photosynthesis is different: it is generally less responsive to changes in temperature and, for C3 plants, it generally reaches a maximum at temperatures below 30 C, sometimes well below (Fig. 7). The reason that photosynthesis is less responsive to temperature is partly due to the fact that some of the key chemistry is photochemical and photochemical reactions are not strongly dependent upon temperature. An additional factor is that carbon dioxide, a reagent in photosynthesis, must dissolve in water to make it to the site of photosynthesis (the chloroplast) and the solubility of carbon dioxide decreases as the temperature increases. Because of this, C4 plants, which have mechanisms to concentrate carbon dioxide, generally have higher temperatures for peak photosynthesis than C3 plants.
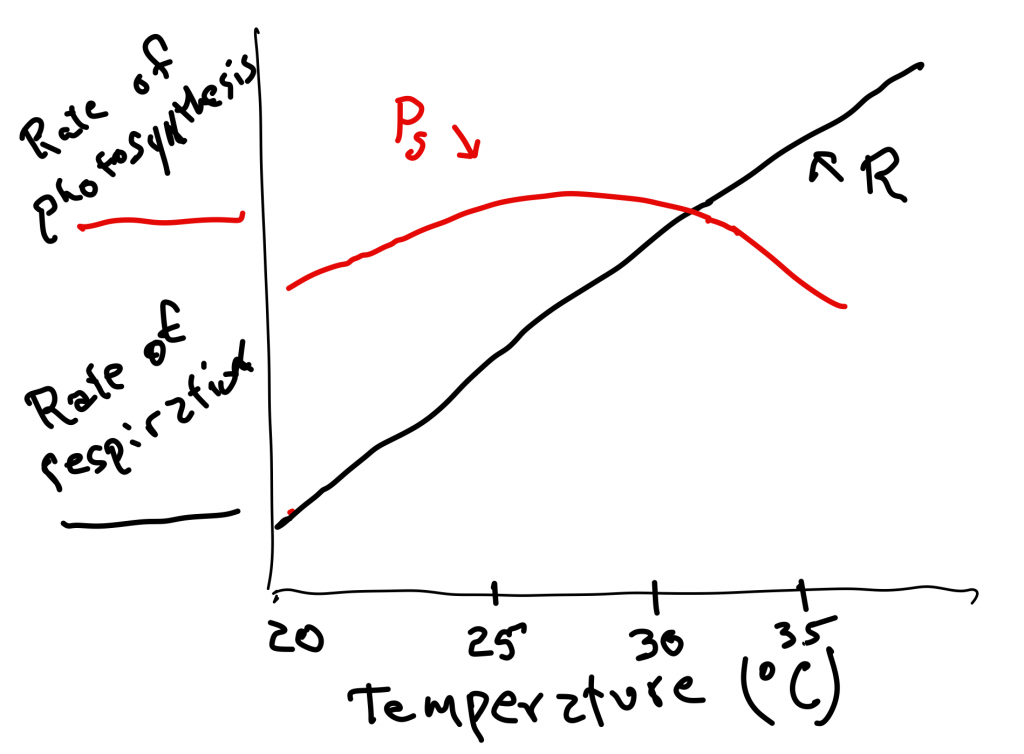
When considering the effects of temperature on photosynthesis, respiration and growth, one can consider photosynthesis as ‘making food’ and respiration as ‘eating food’. As temperature increases in the 25-35°C range, plant appetite continues to increase rapidly while the rate of food production levels off or declines. The net effect of this is that at higher temperatures there is increasingly less food to power new growth. Consequently, plant growth often tails off at temperatures lower than one might expect.
Psychophiles, Cryophiles and Thermophiles
A number of organisms, in particular certain bacteria but also some fungi and protists, are termed ‘psychophiles’ or ‘cryophiles’ because they do best at low temperatures, sometimes at temperatures below freezing. While the basic pattern of increased activity with increased temperature holds, the range of activity is shifted to much lower temperatures: 0 to 10°C and sometimes -10 to 0°C. These organisms have modified membranes and, for the ones that operate below freezing, antifreeze compounds that allow them to operate at such low temperatures. Since a very common mechanism of food preservation is low/freezing temperatures, these organisms may pose problems for the food industry. A cryophilic fungus has turned out to be the culprit in the ‘white-nose disease’ of bats (Fig 8) , a disease that has recently decimated populations of bats that overwinter in caves with temperatures in the 5-15°C range. The bat’s behavior of lowering body temperature during the winter (in order to save energy) has provided these fungi with perfect conditions for growth.
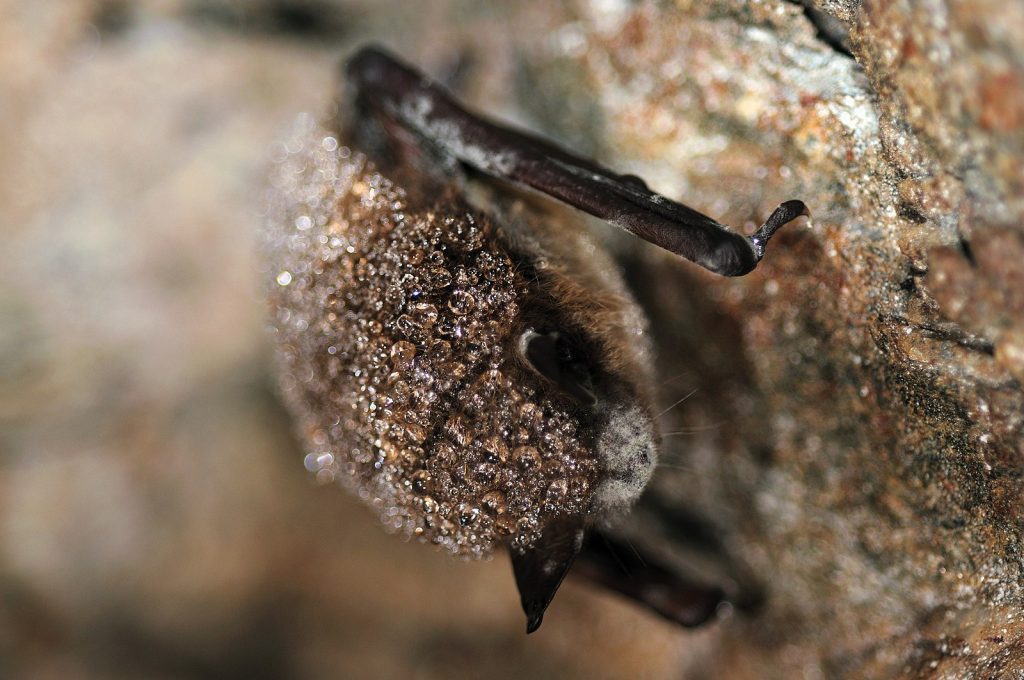
At the other end of the tolerance range are thermophiles, who operate well above the normal activity range of 0-35°C. As with psychophiles, the vast majority of thermophiles are prokaryotes, most often archaea, but there are some thermophilic eukaryotes, all of them fungi. Several of these thermophilic fungi are important components of large-scale composting operations important to the production of commercial mushrooms where the metabolic heat generation of the compost can elevate the temperature of the mulch to 80°C.
Fruit Ripening
One additional process affected by temperature will be mentioned because of its commercial significance: fruit ripening. In addition to the influence of temperature on the growth of fruits, the temperature continues to affect fruits after growth ceases, in the developmental process we describe as ripening. This process brings about important changes in characteristics (aroma, color, taste, texture) that are of significance to both consumers and producers. This developmental process is often controlled in various ways by temperature, and temperature can have a significant impact on the commercial value of a variety of fruit crops by influencing the appearance of both desirable and undesirable traits. In a more general sense, the temperature can influence the ecologically significant characteristics of fruits that affect their role in seed dispersal by influencing their attractiveness to frugivores.
Temperature as a Cue
For many plants, and some fungi and protists, temperature provides an important cue that is used to coordinate growth and other activities with seasonal changes in conditions. Just as gravity organizes plant activity in space, the temperature can coordinate plant activity in time. In this situation, the temperature is not just a condition that the organism responds to, it is a signal that conveys information about what conditions will be like in the future, similar to the way that daylength (photoperiod) can provide information about the coming seasons. However, since the noise in the temperature signal is substantial, using it to predict future conditions is more complicated: a return to warm conditions after cold ones could be a January thaw or it could be a real (spring) thing. For many plants, spring is sensed as a warm period after a measured period of cold. An example of this is seen in the germination patterns of many seeds. The seeds of most plants are shed in a dormant condition. Although some seeds don’t have specific germination requirements, they simply need a few weeks and they are able to germinate (this has been selected for in many crop species), many seeds require specific conditions or a series of specific conditions in order to germinate. Especially in temperate habitats, it is often a cold temperature treatment that will ‘break’ dormancy, i.e., allow the plant in the seed to resume growth (Fig. 9). This pattern is beneficial because it would prevent seeds from germinating and thereby becoming susceptible to cold until after the harsh conditions of winter. Because the original horticultural practices developed to break seed dormancy involved layering seeds and keeping them moist and cool, such treatment is termed ‘stratification’. The key part of the treatment is the temperature treatment, not the layering. Contrary to the normal metabolic pattern, where activity is promoted by warmer temperatures (i.e., warmer temperatures produce more response than lower ones), in the stratification response, activity is promoted by lower temperatures, with colder temperatures having greater effects than higher ones. Typically temperatures need to be below 50°F (10°C) to be effective and become more effective as the temperature decreases down to 0°C. Temperatures below 0°C, and temperature treatments when the seed is dried out, are not effective, indicating that metabolic activity is essential for the response. The process can be modeled in a manner similar to growing degree days, except in this case what is accumulated, termed ‘chill units’, requires temperatures below a certain threshold (typically 10°C), and more chill units are accumulated as the temperature decreases down to 0°C. When a seed accumulates enough chill units its dormancy is broken; at this point, the embryo’s behavior is typical of most organisms, and activity (growth) is promoted by warmer temperatures.
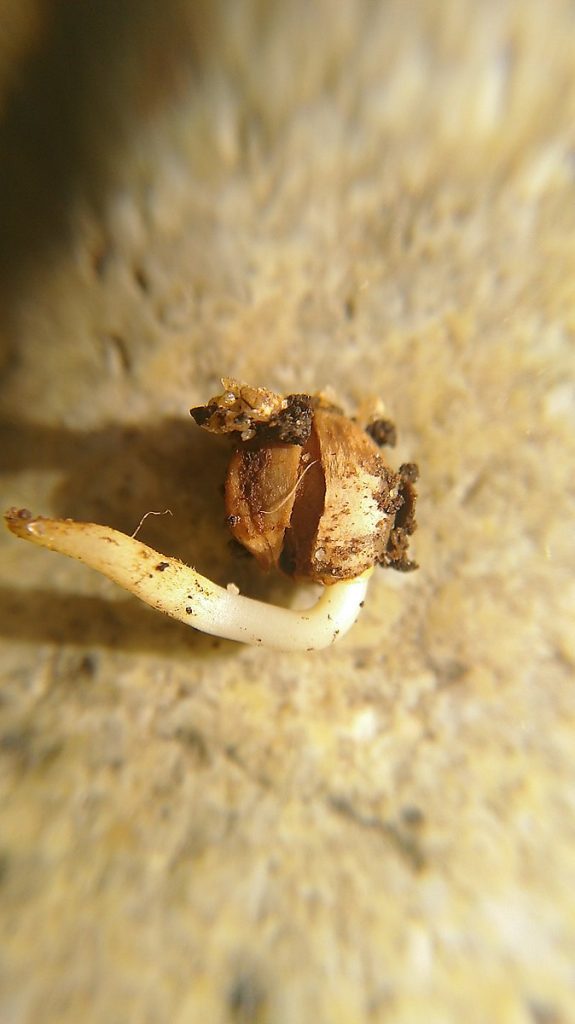
Besides breaking the dormancy of the embryos in seeds, cold treatments are also a very important cue in breaking the dormancy of shoot apical meristems (buds). Home gardeners often bring shoots of flowering trees and shrubs (e.g., apple) indoors to ‘force’ them to flower early (Fig. 10). This practice works as long as the shoot has been exposed to cooler temperatures for a long enough length of time prior to warming it up. Shoots often will not force in December but will in February. The exact amount of ‘chill time’ that a shoot needs before it will respond to warmer temperatures varies. This phenomenon is a reason why certain trees may not be able to be grown in southern latitudes: they never receive enough cool temperatures to cause them to emerge from dormancy.
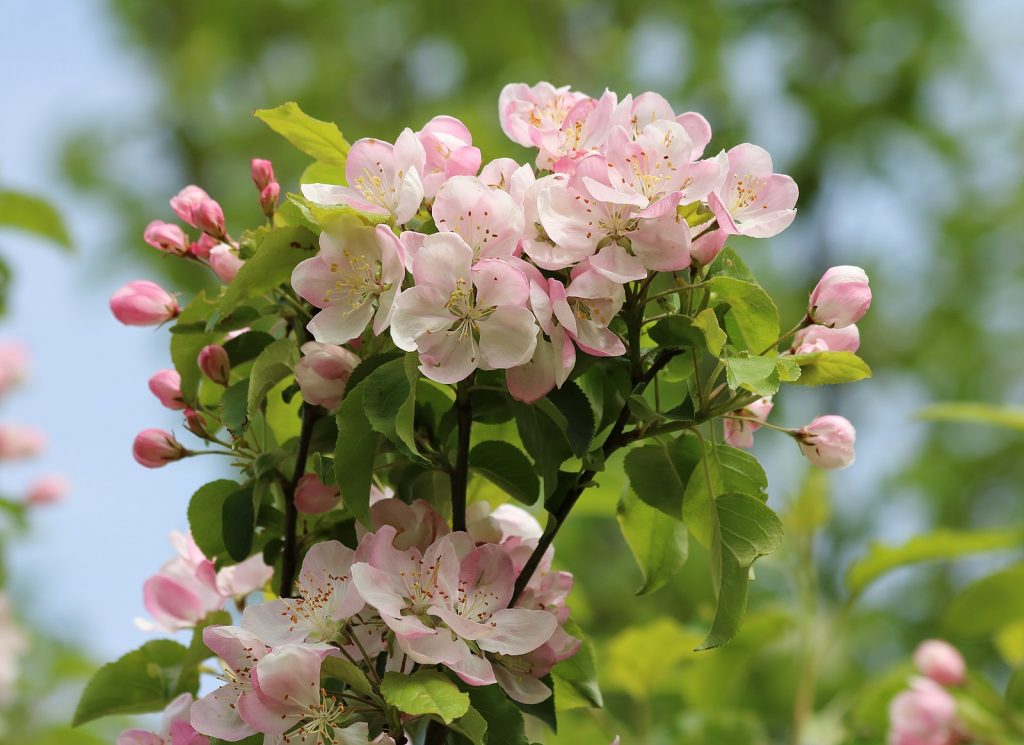
Accumulation of chill units is also a cue for some plants to flower, a process termed ‘vernalization.’ In some situations the cold treatment itself is the trigger for flowering; in other situations, the cold treatment simply flips a switch and allows the plant to flower in response to a second signal (e.g., photoperiod) that previously would not elicit any response.
Temperature’s effect on other conditions
Temperature is also important because it interacts with a number of other conditions. In terrestrial habitats the most significant interaction is between temperature and moisture. Temperature affects evaporation, and occasionally condensation, rates. Evaporation occurs when an individual water molecule is moving fast enough to escape the cohesive forces of its neighbors. Since the velocity of molecules is a function of temperature, the warmer it is the more likely evaporation is to occur. The tendency of a substance to evaporate is reflected in a property called vapor pressure, which measures the amount of the substance (in this case water) present in the vapor state when the liquid and air are in equilibrium. Figure 11 shows the very strong effect temperature has on vapor pressure, reflecting temperature’s ability to influence evaporation.
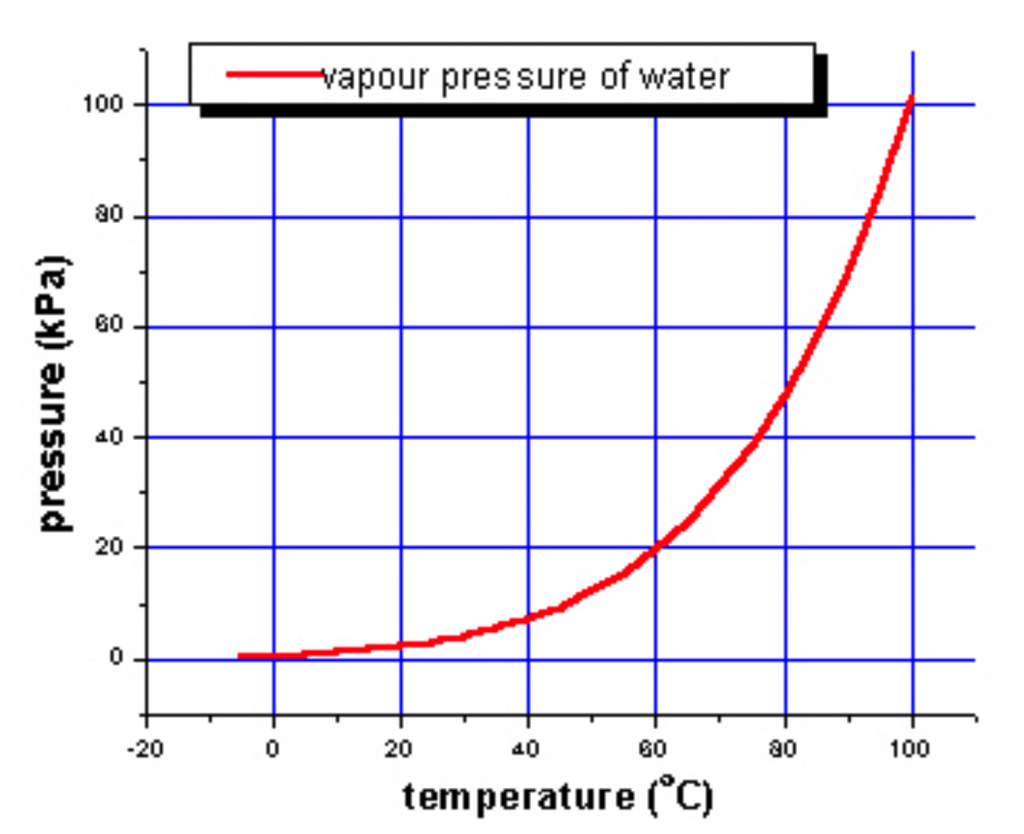
Under certain circumstances, evaporation will be directly related to vapor pressure and consequently would roughly double with each 10oC rise in temperature. In environments where lost water is difficult to replace, temperature can be of great significance to desiccation rates and the survival of organisms. For plants and fungi, the combined factors of temperature and water availability are of utmost importance in determining species abundance and activity. Note that although the temperature has a major influence on evaporation, other factors are also important including the humidity of the air, the surface area of contact between the air and the water, and the degree of mixing (convection) of the air above the hydrated surface. Finally, although temperature affects evaporation, evaporation, in turn, affects temperature. In the case of plant leaves, evaporation can cause leaves to be significantly (over 2o C) cooler than the air temperature.
Temperature also affects the availability of certain compounds by affecting their solubility in water. While the solubility of many solids in water increases with higher temperatures, the opposite is true for gases, and in particular for carbon dioxide and oxygen. This is particularly significant for photosynthesis and, as described above, is part of the reason why the response of photosynthesis with increasing temperature is not comparable to that for respiration even though both involve a host of enzyme-mediated chemical reactions that generally are enhanced at higher temperatures.
Another situation where the decreased solubility of gases at high temperatures can be a problem is flooded soils. The effect of flooding is more damaging at high temperatures than at low ones. Several factors contribute to this: (1) decreased solubility of oxygen at higher temperatures, (2) increased rates of soil respiration (carried out by the sum of organisms present in the soil—plant roots, fungi, bacteria, protozoans, etc.) at higher temperatures. Increased respiration means decreased oxygen (3) increased oxygen requirements for plant roots because of the higher metabolic rates associated with higher temperatures.
Water and Terrestrial Habitats
Along with temperature, moisture is a key environmental variable dictating the distribution of organisms on terrestrial habitats. The reasons for this should be clear:
- water is an essential component of living tissues and a reagent in many essential reactions, including photosynthesis and ATP hydrolysis
- in terrestrial habitats, water is nearly always lost to the atmosphere in the process of evaporation
- for terrestrial autotrophs, who obtain carbon dioxide from the atmosphere, evaporation is all the more likely to occur since these organisms must expose themselves to the atmosphere to acquire carbon
- for plants and animals, water is the medium in which materials move when transported and, for animals, water is the basis for excretion
Tolerance to drying
The vast majority of organisms maintain their moisture conditions at a particular level through the familiar process of homeostasis. Most plants (and most organisms in general) would be considered homiohydric, i.e., they maintain their water levels at a ‘set point’. To do this requires that an organism adjust either water gain or water loss. To a limited extent, plants adjust water gain: when they get drier, the driving force for water absorption is increased and this can result in an increased flux (remember the flux equation!). However, plants have only a fairly limited ability to ‘get drier,’ and when they start to desiccate their most significant adjustments involve reducing water loss rather than increasing water gain. When plants experience water deficits their response is to reduce water loss by: (1) reducing the permeability of the plant to water by closing stomates, and (2) reducing the surface area for loss, generally by shedding leaves. Again, remember the flux equation and how these changes relate to it. Leaf loss is especially obvious in seasonally dry habitats where woody species lose all their leaves and herbaceous species spend the dry season as bulbs or other underground parts, both groups producing and possessing leaves only when conditions are mesic.
These measures to reduce water loss come at the price of reducing a plant’s ability to feed itself. The very significant effect that moisture has on plant distributions reflects the fact that individual, population and species success involves balancing water loss and carbon gain. In some situations, a species may be successful by being able to acquire ‘its own’ water source. Alfalfa and mesquite roots often penetrate deep enough to tap groundwater sources unavailable to other plants. However, a majority of plants in an area share a common water source, the soil, i.e., the roots of many species are occupying the same volume of soil as other species, making the supply of water uniform for most species living in an area. Consequently, conservation by one species or individual only leaves water that can be taken by other species. Species are successful by manag ing their overall growth and patterns of growth. This represents an area of diversification between different species.
Homeohydric organisms are intolerant of desiccation and die if their water status drops below a certain level; most plants cannot recover from a loss of 10-15% of their water. Probably the main reason for this in vascular plants is ‘catastrophic xylem dysfunction’ which is a consequence of cavitation and positive feedback loops related to cavitation. Recall that individual vessels and tracheids may cavitate if the water in them is pulled too forcefully, the result of water loss at a time when there is a restriction of water acquisition as the soil dries. Cavitation results in a loss of part of the xylem conducting system and this makes cavitation more likely in the remaining conducting elements (see the flux equation): increased resistance to flow as a result of cavitation means that there has to be a greater pull (higher tensions) in order to acquire the same amount of water. Thus there is a positive feedback loop: cavitation makes more cavitation likely which will make more cavitation likely. Catastrophic xylem dysfunction (no ability to transport water) may result quickly after the first cavitation event, leaving the plant with little to no ability to rehydrate itself.
There are a small number of organisms, including a very few animals (tardigrades), some protists, and a few plants, that are poikilohydric: their water status is not strongly regulated but is allowed to assume the level dictated by the environment that they are in. Obviously, these organisms can only be successful (assuming that they live in an environment that dries out at least some of the time) if they are tolerant of desiccation, a phenomenon that is very rare in organisms, although they may produce parts like spores and seeds that are tolerant. Poikilohydric organisms can lose up to 90% of their water yet are still able to revive themselves when water again becomes available. The only plant groups where desiccation tolerance is common are in the non-vascular plants, in many mosses and some liverworts (ironically groups that are often considered to be restricted to moist environments). Desiccation tolerance is also present in a few clubmosses (Fig 12, 13) and ferns (Fig 14, 15) and in a very few seed plants.
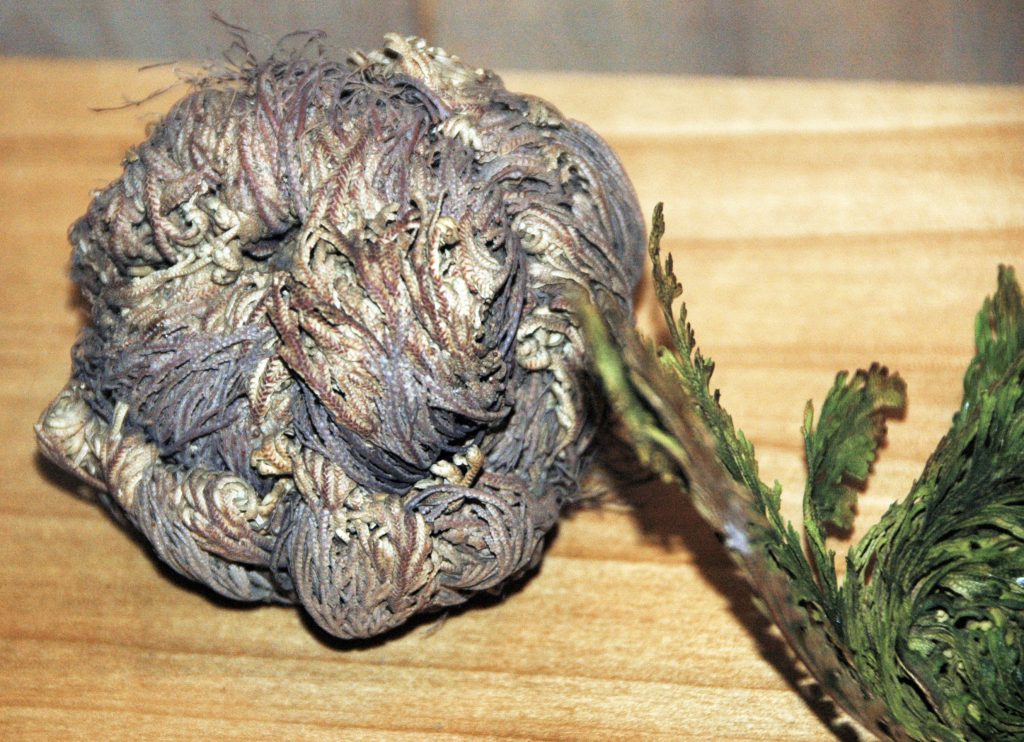
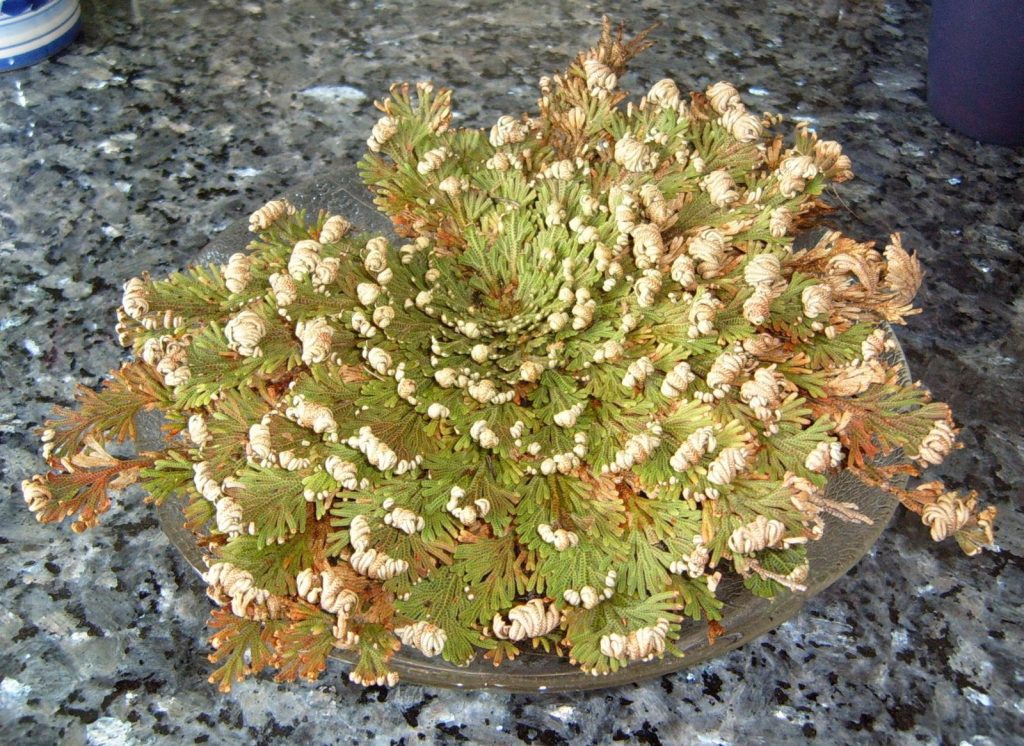
While there are some mosses that are restricted to moist habitats, most are desiccation-tolerant and many are particularly prominent in arid situations, both arid habitats (deserts) and arid portions of more mesic habitats, e.g., growing on rocks that have no ability to store water. Some of these species do have features to lessen water loss, e.g., a drying response that involves coiling of ‘leaves’ around the stem, lessening the surface area exposed to the atmosphere. Nonetheless, many mosses are capable of tolerating extreme desiccation and the whole organism, not just a select part, can remain viable after undergoing repeated cycles of desiccation. They are inactive during dry periods but are able to quickly resume activity when moistened.
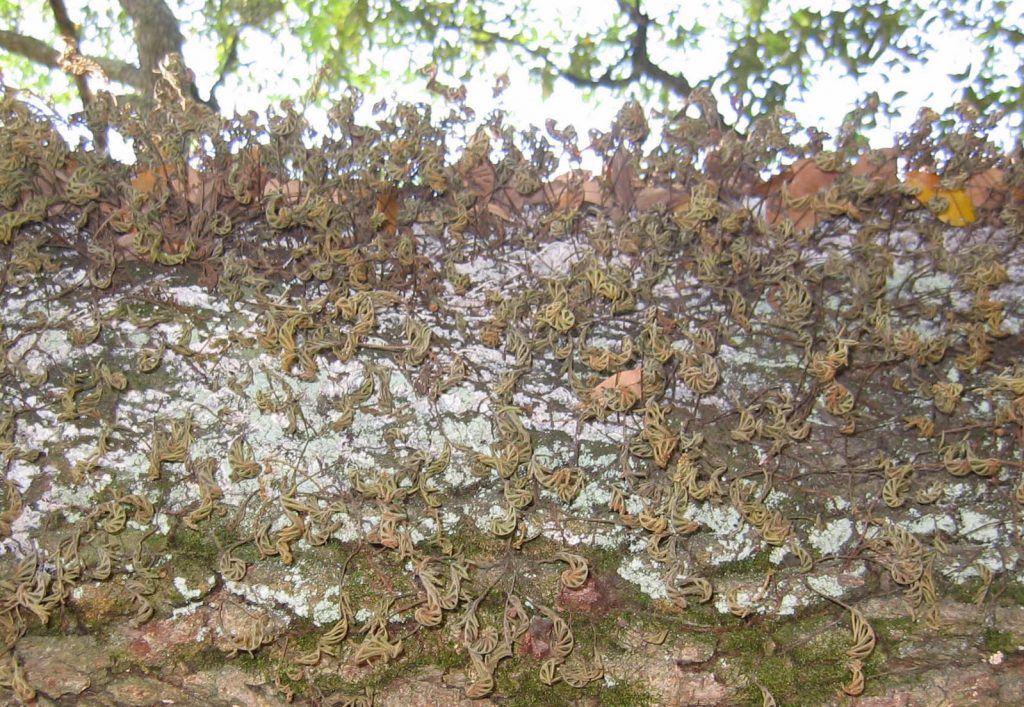
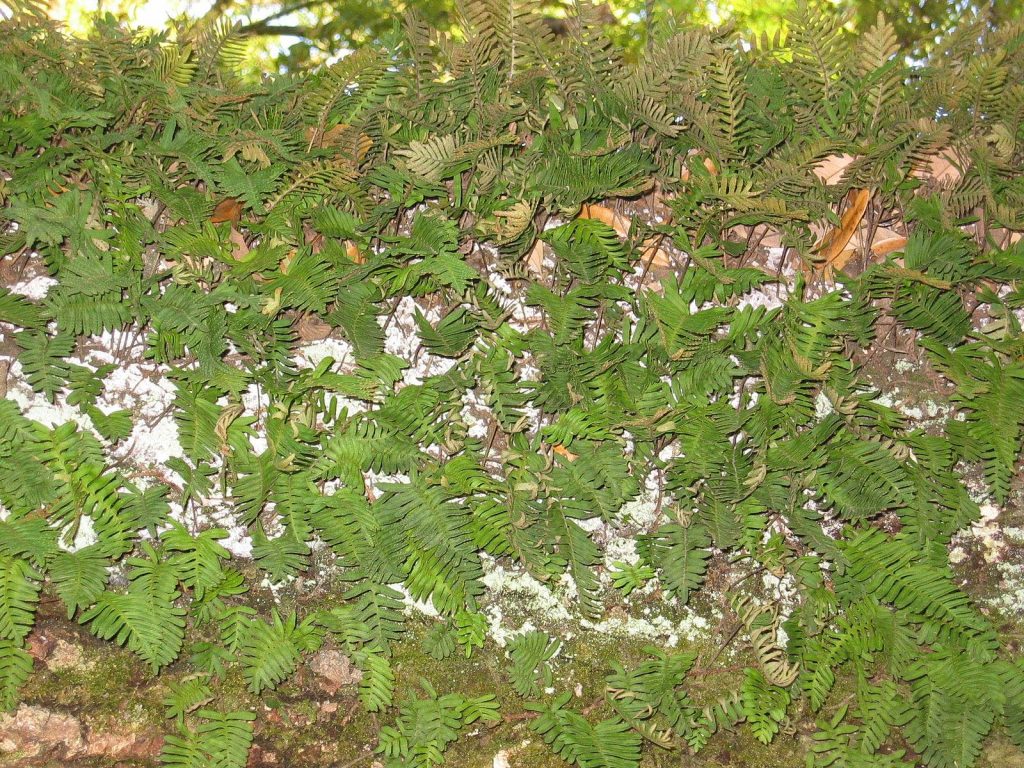
Tolerance of flooding
At the other extreme, terrestrial organisms can be affected by excesses of water. Generally this is an indirect effect of a lack of oxygen that was discussed above when considering the interactions between temperature and oxygen.
Water in Aquatic Environments
Salinity
One might assume that there are no problems associated with water in an aquatic environment since water is abundant. However, water does play an important role in dictating the organisms present in any particular aquatic environment, primarily because of the process of diffusion. Because water tends to diffuse from regions where it is purer to regions where it is less pure, living things are strongly affected by the purity of the water that they live in, and this is primarily determined by the water’s salinity. All life has the ability to both accumulate and to generate solutes, and therefore the water in organisms is decidedly impure. Consequently, if organisms are placed in pure water, water diffuses into them. The influx of water has two potential results: one is chemical, the cell solutes become so dilute that normal functioning is impeded; the second is mechanical, the influx of water can cause the organism to swell and ultimately burst. However, if the organism is enclosed in a rigid container the influx of water pressurizes the organism and the flow of water ceases with only modest changes in volume. Another possibility that allows organisms to live in ‘fresh’ (i.e., pure) water is to have mechanisms that allow water to be expelled from the organism at the same rate that it enters.
The problems associated with the diffusion of water into organisms are eliminated if the purity of water is the same outside as it is inside the organism, and there are a number of organisms (many marine animals, some protists) that only live in such an environment, where the purity of the cytosol is comparable to that of seawater.
There also are habitats, termed saline (‘salty’, although the salt need not be NaCl) habitats that have a water purity even less than that of most organisms. Most forms of life are excluded from such saline environments because diffusion causes them to lose water and they cannot tolerate the decrease in the internal water content that results from water loss. Organisms that are able to tolerate such environments do so by having more than the normal concentration of solutes in their cytosol, generally adding unique solutes that are typically not found in living things.
The problems of life in saline environments are not restricted to reduced water content. Additional problems stem from toxic concentrations of the solutes that make the habitat saline. Generally, this would be sodium and chloride ions but there are habitats where other solutes (e.g., potassium, calcium) are damaging. With the exception of sodium, which most plants don’t require, these elements are essential for living things, but at high concentrations they can interfere with normal cellular or organismal functioning and become toxic.
Oxygen and Aquatic Environments
Oxygen is of key importance in aquatic environments. Oxygen readily dissolves in water but its solubility is such that oxygen is generally less available than in terrestrial habitats. More significantly, its abundance varies much more in aquatic habitats than in terrestrial ones. In terrestrial environments the oxygen levels of the air rarely change much because of convectional mixing (winds), due to the much lower density of air compared to water, and, to a lesser extent, due to the much more rapid rate of diffusion in gases. Processes (primarily biotic ones) may increase or decrease oxygen levels slightly but rapid mixing with the huge reservoir of oxygen in the atmosphere as a whole maintains the concentration of oxygen in the air at the normal values of slightly below 20%.
In contrast, liquid water is much denser, its movement is much more sluggish and consequently, the possibility of localized areas in aquatic habitats with varying oxygen concentrations is much more likely. Under certain situations aquatic habitats will be saturated with oxygen, i.e., holding all the oxygen that can dissolve in water, an amount that is temperature-dependent and decreases with increasing temperature. As would be expected, water is saturated with oxygen when the air and water are in close contact (i.e., the surface of bodies of water) or in situations where photosynthetic rates are high, thus increasing oxygen levels (generally these areas are also close to the surface). However, there are lots of aquatic situations where the amount of dissolved oxygen is not at saturation. Generally, this is due to the depletion of oxygen by living things and the lack of mixing with water that is in contact with the air, a situation found at the bottom of lakes, in slow-moving streams, and waterlogged soils. Impediments to mixing will make oxygen depletion more likely. One of the most common impediments are water density gradients, the result of temperature differences. Less dense water ‘floats’ on more dense water and this will reduce oxygen transfer from surface waters to the denser water below. The density of water decreases as the temperature increases from 4 C; hence, in this range, warmer water is less dense than cooler water and a consequence of this is that the water is layered (‘stratified’) during the times of the year that it is being heated from above, during the summer in northern latitudes, all year round in the tropics. The wind may cause turbulence in the water column and mixing but the penetration of mixing is limited by the density differences. The greater the temperature differences between the top and bottom, the more resistant the lake is to mixing. Because of this stratification, the bottom layers of a lake may become depleted of oxygen because they do not mix with the oxygenated water at the surface. In tropical areas, low oxygen levels at the bottom of lakes occur year-round, but in temperate areas, mixing, and as a result aeration of the bottom layers, becomes increasingly likely during the fall. This is because, unlike the heating of the summer, which reinforces the stratification by making the top warmer, cooling breaks down the stratification by making the surface denser. As long as the lake is in a location that cools enough, the lake will eventually become ‘isothermal’, i.e., the same temperature throughout the water column. Being isothermal also makes the lake the same density from top to bottom and, as long as the wind blows hard enough (how hard depends on the depth of the lake), the lake will ‘mix’, bringing aerated water from the bottom to the top. This is significant from a nutritional standpoint because organic material settles to the bottom of the lake and decomposes there. Minerals released by decomposition are only distributed throughout the water column when the lake is mixed. Further cooling of the lake in late fall (northern hemisphere) once again results in stratification, as the density of water decreases (and the colder water floats on top) when the temperature drops from 4 to 0 C. If the lake freezes, the addition of an ice layer eliminates any wind-driven mixing whatsoever and oxygen levels at the bottom of the lake once again drop if respiration exceeds photosynthesis. This is usually the case (although some photosynthesis does take place in ice-covered lakes, it is relatively low because of decreased light penetration). In the spring (in the northern hemisphere) heating of the surface first eliminates the ice and then eliminates the stratification, making the lake uniform in temperature and density at 4 C, and again allowing for mixing by the wind. Further heating causes the lake to again become stratified. Thus lakes in temperate habitats have an annual cycle that includes two brief periods in the fall and spring where mixing is very likely, so long as the wind blows and the lake is not too deep. This situation, termed spring and fall ‘turnover’, is highly significant because it allows for mixing of the water column, bringing oxygenated water to the bottom of the lake (and also bringing minerals to the top of the lake (see below).
Another hindrance to water mixing is material that restricts water movement. Sphagnum bogs usually have oxygen -depleted water at very shallow depths of a few centimeters, depths that usually receive ample oxygen from the close contact with the atmosphere because of mixing caused by even slight winds. However, a sphagnum mat hinders the movement of the water column and although the oxygen-rich atmosphere is centimeters away, oxygen levels drop to nearly zero at very shallow depths. A similar thing can happen in ponds that are covered with a thick ‘pond scum’ of algae. The low oxygen conditions hinder decomposition and result in an accumulation of organic material (‘peat’) and low levels of nutrients.
Light and Aquatic Environments
As is the case in terrestrial environments, light plays an important role in dictating the distribution of photosynthetic organisms. It is even more important in aquatic environments because significant differences in oxygen concentrations can develop within the water column, depending upon the balance between photosynthesis and respiration. Light intensity is influenced by the depth and transparency of the water, the latter often being strongly influenced by the number of living things living in the water. Since aquatic photoautotrophs need light to survive, they need to be in the upper levels of the water, or, in the case of some flowering plants, float on the surface or even emerge from it. Phytoplankton (small photosynthetic organisms that ‘float’ in the water column) need to have features that prevent their settling. Features that are sometimes significant are large surface area to volume ratios (i.e., not spheres), oil or air bodies that decrease density, flagella and phototaxis (movement towards light, see cryptophytes). Some phytoplankton are known to migrate up and down diurnally, moving up to gain more light during the daylight hours.
Currents and Aquatic Environments
Currents are very significant in some aquatic environments and can have a strong influence on community structure. Currents are significant because they can mix portions of the water column, in particular, they can bring oxygen from the upper layers to the lower layers and nutrients from the lower layers to the upper layers. This is significant because oxygen may be limiting in the lower layers and nutrients may be limiting in the upper layers. Consequently, the current can substantially change the activities taking place.
Nutrients and Aquatic Environments
Nutrients are often limiting primary productivity and consequently total activity in aquatic situations. This is a result of the fact that most aquatic environments have two distinct parts: the upper layers where photosynthesis occurs and where inorganic nutrients are incorporated into biomass, and the bottom region, where there is typically little photosynthesis, but where biomass from the upper layers tends to settle, decomposition occurs, and nutrients are released. In such a system the activities of both the top and bottom can be limited by a lack of interaction between the two parts: the upper layers are limited by a lack of nutrients, hence little accumulation of biomolecules occurs; the bottom layer becomes limited by a lack of material to eat.
Nutrients transported into aquatic systems can be very important. Nutrients can be carried into aquatic systems by runoff from the surrounding land, especially agricultural land where nutrients are added through fertilization. Nitrogen and phosphorus often play key roles in determining the amount of primary production occurring and the total amount of biological activity taking place. However, as was the case in terrestrial systems, there are circumstances where other nutrients can play key roles.
Further Reading and Viewing
- “How Does Deep Water Rice Solve Its Aeration Problem” by Ilya Raskin and Hans Kende. How rice solves its aeration problems.
- “Mushrooms as Rainmakers: How Spores Act as Nuclei for Raindrops” by Maribeth O. Hassett et al. Mushrooms as rainmakers.
- “Deciduous Trees Allow Higher Seasonal Water Yields: Flowering Plants” by Leon Wang. Deciduous tree influence on water storage in forests.
- “Hydraulic lift: a potentially important ecosystem process” by JL Horton et al. Hydraulic lift, an interesting plant induced water flow.
- “Horizontal transfer of an adaptive chimeric photoreceptor from bryophytes to ferns” by Fay-Wei Li. An amazing evolutionary story of horizontal gene movement related to adaption in low light environments.
Media Attributions
- Sugar Maple © Superior National Forest is licensed under a CC BY (Attribution) license
- Sugar Maple DIstribution © Elbert L. Little, Jr., USGS is licensed under a Public Domain license
- Northern Hardwood Forest © Nicholas A. Tonelli is licensed under a CC BY (Attribution) license
- Membrane © Mariana Ruiz is licensed under a Public Domain license
- PEP © Tims2015 is licensed under a CC BY-SA (Attribution ShareAlike) license
- Ergot © JoJan is licensed under a CC BY-SA (Attribution ShareAlike) license
- Bat © U.S. Fish and Wildlife Service Headquarters is licensed under a CC BY (Attribution) license
- Orange seed © Eiku is licensed under a CC BY-SA (Attribution ShareAlike) license
- dry clubmoss
- Rose von Jericho © Fabrizio Cortesi is licensed under a CC BY-SA (Attribution ShareAlike) license
- PoliypodiumPolypoioides © DanielCD is licensed under a CC BY-SA (Attribution ShareAlike) license
- Green Polypods © DanielCD is licensed under a CC BY-SA (Attribution ShareAlike) license