Chapter 20: Photosynthesis
Plants are literally formed ‘out of thin air’. While a small portion of their (dry weight) mass comes from the soil, approximately 98% comes from the atmosphere. A typical serving of potatoes is 6 oz = 170 grams. Since potatoes are roughly 80% water a serving of potatoes is about 34 grams of dry weight. This weight is nearly pure starch which has a chemical formula (C6 H12 O6). Carbon atoms represent 40% of the weight of starch (or of any hexose or hexose polymer) so that 34 grams of potato has 13.6 grams of carbon = 1.13 mols of carbon. This has been derived from the air which is roughly 400 ppm carbon dioxide. In order to acquire the carbon needed for a serving of potatoes the plant has to extract carbon from approximately 67 thousand liters of air. This photosynthetic ability is even more remarkable because it involves a transfer of the energy of light (electromagnetic radiation) into chemical energy present in the carbohydrate that is produced. The synthesized hexoses have considerably more energy than the raw materials (carbon dioxide and water) used to produce them.
TOPICS
- Overview
- The light dependent reactions
- The carbon dioxide assimilation reactions
- Photosynthetic constraints: the photosynthesis/transpiration compromise
- C4 photosynthesis
- CAM photosynthesis
Overview
Photosynthesis produces carbohydrates. The name reveals what they were originally considered: hydrated carbons (i.e., water added to carbon), but research has revealed that a more accurate description of carbohydrates is reduced carbon dioxide. This is illustrated in the overall equation for photosynthesis:
CO2 + H2O —> (CH2 O)n + O2
Hydrogen is added to the carbon dioxide, i.e., it is reduced, and hydrogen is being removed from oxygen, i.e., it is being oxidized. Note that, as in the case for cellular respiration, the overall equation is a summary of the net effect of multiple reactions taking place simultaneously. Specifically in photosynthesis, carbon dioxide does NOT react with water. Instead, both water and carbon dioxide are consumed in a group of reactions that ultimately produces oxygen and carbohydrates.
The overall reaction as written is exceptional because oxygen is a highly electronegative atom, one that attracts hydrogens strongly, much more strongly than the carbon atom that the hydrogens are transferred to. For this reason, the reaction is ‘uphill’ and unlikely to occur, while the reaction in the opposite direction is much more likely to occur, with carbohydrates being oxidized by oxygen to produce carbon dioxide and water (see the previous chapter). The last chapter illustrated that cellular respiration (carbohydrate oxidation) is ‘driven’ by the electronegativity of oxygen; this electronegativity ‘pulls’ electrons through the inner mitochondrial membrane, ultimately uniting them with oxygen. Thus a significant question concerning photosynthesis is what pulls electrons away from the oxygen of a water molecule—what has a stronger ‘pull’ for hydrogens than oxygen (i.e., what is a more powerful oxidant than oxygen)? Light interacting with the pigment chlorophyll plays a critical role in generating the strong oxidant. Also critical is the structure of the chloroplast which, like mitochondria, consists of a complex structure of membranes separating aqueous compartments. The membranes contain chlorophyll (Fig. 1) as well as other pigments and proteins, organized in very specific ways.
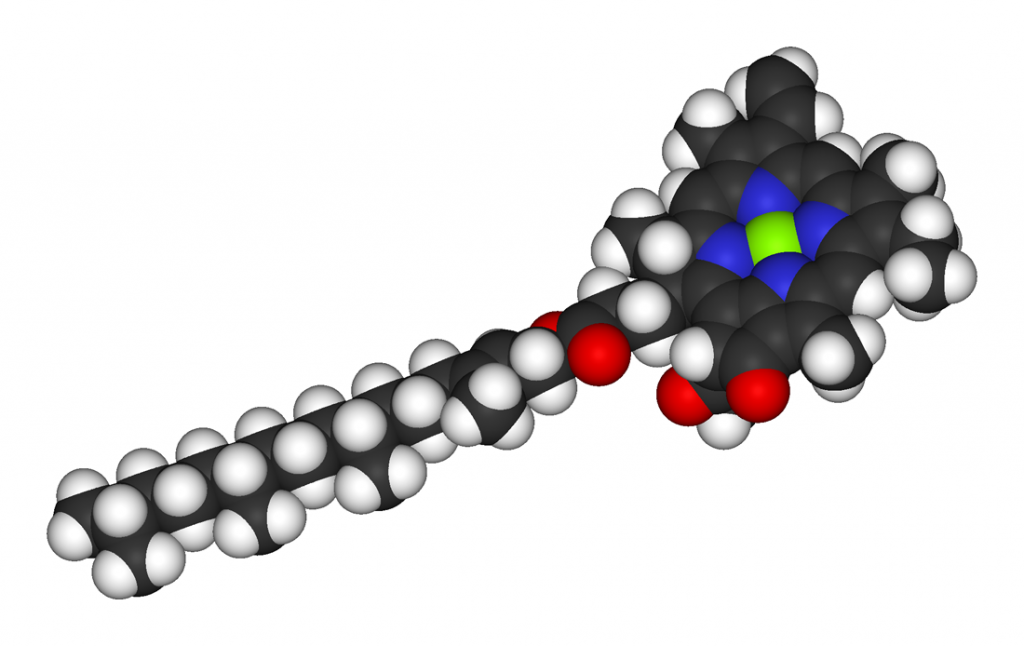
The light-dependent reactions of photosynthesis
Light’s role in photosynthesis is in rearranging specific chlorophyll molecules, causing them to lose electrons (oxidizing them) and thereby making them oxidants that remove electrons from other molecules and ultimately from water. Light is a form of energy and consequently is able to change the circumstances of the material that it interacts with. In particular, light changes the electron configuration of chlorophyll, shifting an electron from its normal position to a situation where it is more likely to escape from the chlorophyll molecule, i.e., after absorbing light, the chlorophyll is more likely to be oxidized. The oxidation of chlorophyll is made even more likely because there is a molecule nearby in the inner membrane of the chloroplast that is capable of accepting the electron. The electron lost from chlorophyll ultimately (after many steps) ends up associated with the carbon of a carbon dioxide molecule, forming carbohydrates. The oxidized chlorophyll molecule (i.e., the one missing an electron) is not a strong enough oxidant to extract electrons from water. But it can act on the ‘oxygen evolving complex’, an enzyme complex that contains four manganese atoms. A chlorophyll lacking an electron is capable of oxidizing one of the four manganese atoms. After this process is repeated three more times and all four of the manganese atoms are oxidized, the molecule is now a strong enough oxidant to act on two water molecules, removing four electrons, one each from the four hydrogens, and these replace the electrons that had been lost from each of the four manganese ions. This process produces four protons (H+) and one molecule of O2.
The electron lost from chlorophyll follows a path through a membrane similar to the flow of electrons through the inner mitochondrial membrane in the process of ‘electron transport’ (= oxidative phosphorylation) of cellular respiration, sometimes utilizing similar electron carriers.
And, similar to some of the steps in oxidative phosphorylation, some of the electron transfers have the effect of moving protons from one side of the membrane to the other. Moreover, the ‘splitting’ of water, performed by the manganese-containing protein, adds protons to that same side of the membrane. The accumulation of protons on one side of the membrane creates an electrochemical gradient across the membrane. And, because of the electrochemical gradient, ATP can be synthesized as it is in the electron transport chain of cellular respiration. But in this case the electrons are not flowing to oxygen. They move first to a different chlorophyll atom, but only after it, like the chlorophyll molecule described earlier, has been oxidized by the action of light. When this second chlorophyll receives an electron from the electron transport chain it is converted back to its normal state, and in this state the chlorophyll can once again absorb light, be excited, and lose an electron, thus continuing the process. Note that we have identified two distinct chlorophyll molecules, both of which absorb light and lose electrons. One chlorophyll molecule (called chlorophyll 680) obtains ‘replacement’ electrons from a manganese containing protein; the second chlorophyll (called chlorophyll 700) obtains replacement electrons from the electron transport chain. The flow of electrons described so far is:
water — >
manganese enzyme complex — >
chlorophyll 680 — >
electron transport chain — >
chlorophyll 700.
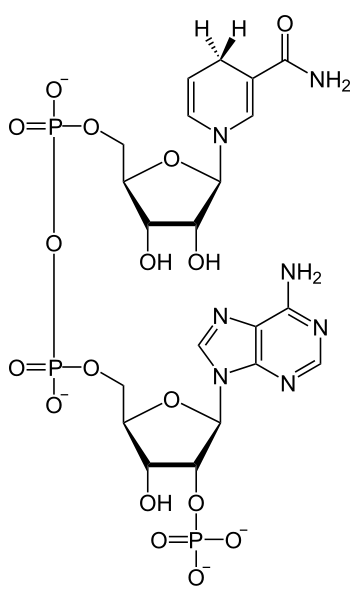
But they haven’t finished their journey yet! Ultimately these electrons will be reducing carbon dioxide, but before getting to the carbon of a carbon dioxide they are transferred to another important intermediate, NADP+ (Fig. 2) , a compound very similar to the NAD+ that operates in the mitochondria during cellular respiration. Like NAD+, NADP+ can accept two electrons and a proton to form NADPH and can lose the same elements to reform NADP+, i.e., it is an electron carrier that (as NADP+) can oxidize compounds and (as NADPH) can reduce compounds. NADP+ receives electrons from carrier molecules that receive them from an ‘excited’ chlorophyll 700 molecule. NADPH is a relatively stable molecule and is water-soluble, unlike many of the electron carriers involved in photosynthesis that are soluble only in the lipids of the chloroplast membrane.
In total, what we have described so far is a light-driven flow of electrons from water to NADP+, forming NADPH along with O2 (Fig 3). The flow occurs in the inner chloroplast membrane and involves two steps where the energy of light is significant in making electron transfer (i.e., redox reactions) more likely. The flow of electrons through a membrane is capable of creating a proton gradient, as it does in the inner mitochondrial membrane. And, as is the case in mitochondria, this gradient in protons can be used to synthesize ATP. This group of reactions, powered by light and creating NADPH and ATP from NADP+, ADP and inorganic phosphate, is called ‘the light reactions’ and is summarized as:
H2O + NADP+ + ADP + iP —> NADPH + ATP + O2
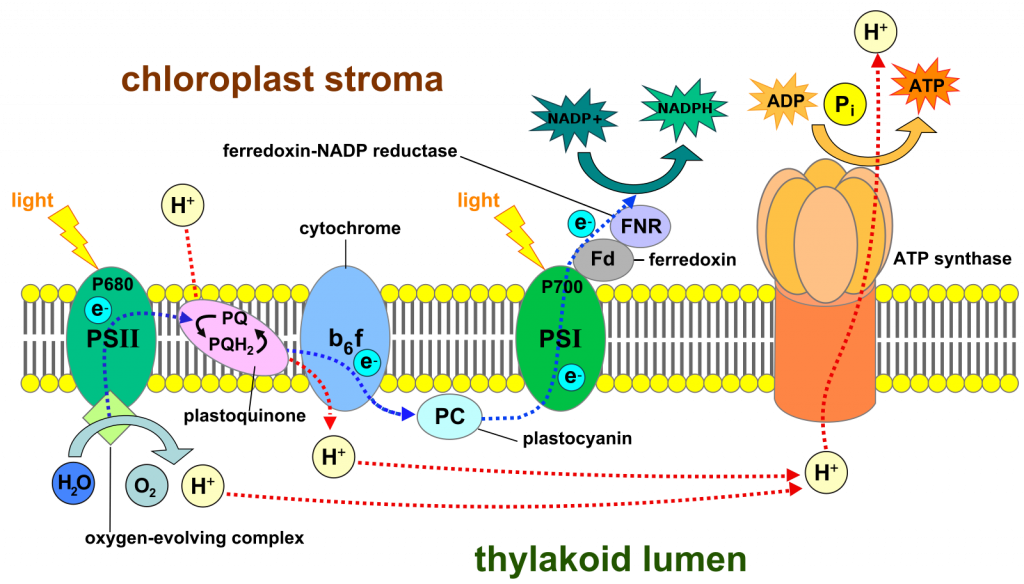
The carbon dioxide assimilation reactions of photosynthesis
The products of the light reactions, NADPH and ATP, are used to synthesize carbohydrates from carbon dioxide, a process called carbon dioxide fixation.
Carbon fixation fundamentally involves the use of the ‘reducing power’ of NADPH to reduce carbon dioxide, and the process is summarized in the following equation:
NADPH + ATP + CO2—> (CH2 O)n + NADP+ + ADP + iP
Note that one of the extremely important aspects of these reactions is that it regenerates metabolites needed in the light reactions: NADP+, ADP and iP. Since the supplies of these metabolites are limited, it is critical that they be recycled. The reactions of carbon fixation can be summarized in three steps: carboxylation, reduction and regeneration.
Carboxylation
Carboxylation describes the incorporation of carbon dioxide into an organic molecule. Interestingly, this can be accomplished without involving any of the products of the light reaction. Carboxylation occurs when carbon dioxide is added to a metabolite called ribulose bisphosphate (RuBP), a five-carbon sugar with two phosphates, in a reaction catalyzed by an enzyme called ribulose bisphosphate carboxylase (rubisco). The resultant 6-carbon compound rapidly breaks down to two molecules of a three-carbon compound called phosphoglycerate (PGA).
Reduction
Although carbon dioxide has been assimilated, the PGA is not a very useful compound because it is too oxidized. To be useful the PGA needs to be reduced. It can then be used as a precursor molecule to make a variety of biomolecules such as sugars, amino acids, nucleic acids and many others. In addition, the reduced compound can be used to make more RUBP and thus allow more carbon dioxide to be assimilated.
The reduction of PGA is accomplished using NADPH and ATP produced in the light reactions of photosynthesis and produces a three-carbon sugar called glyceraldehyde-3-phosphate (G3P).
PGA + NADPH + ATP — > G3P + NADP+ + ADP + iP
Regeneration of RuBP
In order to sustain photosynthesis the plant needs to regenerate RUBP, the 5-carbon sugar that is used to acquire CO2. This occurs when RUBP is synthesized from G3P. Obviously, you can’t make a five-carbon sugar out of a three carbon sugar. You might do it using two G3P molecules but there would be one ‘fixed’ carbon leftover. However, the synthesis can be accomplished tidily if one starts with five G3P molecules (fifteen total carbons) and makes three RUBP’s (also 15 carbons). These reactions are called the Calvin-Benson cycle and they require one ATP made in the light reactions for each RUBP produced.
At the same time, G3P can be used to make six-carbon sugars, in particular glucose and fructose and from them, sucrose, starch, cellulose and a wide variety of polysaccharides.
Putting both these activities together, if six molecules of carbon dioxide are fixed by carboxylating six RUBP’s, then 12 G3P can be produced after reduction utilizing 12 NADPH and 12 ATP. Ten molecules of G3P can be used to regenerate the six RUBP’s and this process requires six more ATP. The remaining two molecules of G3P can be used to form a fructose or a glucose. This is how all plants carry out photosynthesis. Each carbon dioxide assimilated requires two NADPH and three ATP.
Note that ALL of the above equations are summaries of multiple reactions occurring simultaneously. There is a great deal of chemistry taking place in chloroplasts, although the net effect can be expressed simply as
6 CO2 + 6 H2O —> (C6H1206) + 6 O2
Note that the net equation does not include ATP, ADP, iP, NADP+, NADPH, RuBP, PGA or G3P. All of these reagents are produced at the same rate that they are consumed. The net equation also hides the fact that that actually 12 H2O are needed as reactants and that 6 H2O are products (the net effect is simply the consumption of 6 H2O). Part of the elegance of photosynthesis is that in spite of the myriad of reactions taking place, the net effect is very simple. Another aspect of its elegance is that the product of the process, carbohydrate (CH2 O)n, can be used to make all of the diverse chemicals that the plant produces, not just the obvious ones (starch, cellulose, hemicellulose, pectins) but the less obvious ones (amino acids, nucleic acids, lipids, lignin, etc, etc). Moreover, carbohydrates are also used as a source of energy to power these synthetic reactions: In cellular respiration. carbohydrate is consumed to produce carbon dioxide and water while producing ATP and NADH, chemicals that are needed in many of the synthetic reactions.
Carbon dioxide acquisition, problems and solutions
For terrestrial auatotrophs, the carbon dioxide needed for photosynthesis comes from the atmosphere and it is highly significant that very little carbon dioxide is present there; atmospheric carbon dioxide concentration is only 400 ppm — for every million air molecules of air only 400 are molecules CO2. Carbon dioxide enters the leaf by diffusion. Recall the flux equation introduced in Chapter 3 that the flux of CO2 into the leaf is dependent upon the driving force, the difference in CO2 concentration between the inside of the organism and the outside. The outside concentration is set at a low value, 400 ppm, and the inside value for most photosynthetic organisms (for most autotrophs, i.e. plants, this is the intercellular air inside the leaf) is about 100 ppm. This is because rubisco, the enzyme that catalyzes the ‘fixation’ of carbon dioxide, loses the ability to function when CO2 concentrations are lower than 100 ppm. Because the driving force for carbon dioxide diffusion into the leaf is low, the inward movement of carbon dioxide (i.e., photosynthesis) is limited. Again examining the flux equation, it can be seen that flux can be enhanced if the resistance to CO2 entry is low. In the case of a leaf this would mean making the leaf more porous, more open to the outside air. In moist environments, where water is readily available, there is no problem with have very porous leaves (i.e., open stomates), but when water is scarce the plant must balance carbon gain with water loss. Stomatal behavior reflects this compromise: they generally close at night, when photosynthesis is impossible, and during times of drought, when acquiring water to replace that which has been lost is difficult.
The additional carbon dioxide in the atmosphere in the last 200 years probably accounts for the observation that the earth is ‘greener’ (increased leaf cover) now than 50 years ago. More carbon dioxide can act as a ‘fertilizer’ and/or allows plants to survive under conditions of low water supply.
While there are a number of features that allow some plants to survive under dry conditions there is one that directly involves the photosynthetic reactions discussed above. This modified photosynthetic pathway, called the C4 pathway, allows some plants to acquire carbon dioxide while losing less water than a normal plant would. C4 plants concentrate carbon dioxide in a relatively small portion of the leaf, called the bundle sheath cells. The pathway utilizes two carboxylations. The first occurs in leaf mesophyll cells and utilizes an enzyme, PEP carboxylase, that adds a carbon to a three carbon compound, phosphoenolpyruvate (PEP). PEP carboxylase can operate at carbon dioxide levels down to around 10 ppm, roughly 1/10th of the concentration needed for rubisco to operate. The four carbon compound produced by PEP carboxylase is transported via plasmodesmata to a sheath of enlarged cells (Fig. 4) that surrounds vascular strands of the leaf. Here the four-carbon compound is decarboxylated, releasing carbon dioxide that is subsequently fixed in normal photosynthesis using rubisco. The remaining three carbon fragment is transported back to the mesophyll cells. Using this system of two carboxylations allows plants to produce an environment (the bundle sheath cells) where there is a higher concentration of carbon dioxide. It is only in this location where rubisco is present. Because of the CO2 concentrating mechanism, the [CO2 ] can be greater than 100 ppm in the bundle sheath cells while the [CO2 ] of the air inside the leaf approaches 10 ppm. Because of the lower concentration of CO2 inside the leaves of these plants there can be a greater driving force for diffusion into the leaf. This allows the resistance to be higher (stomates more closed) while still achieving the same amount of carbon dioxide flux (photosynthesis) as a plant that didn’t utilize this pathway. Thus the leaf can function photosynthetically while being much less porous to carbon dioxide, thereby losing less water in transpiration than a ‘normal’ plant.
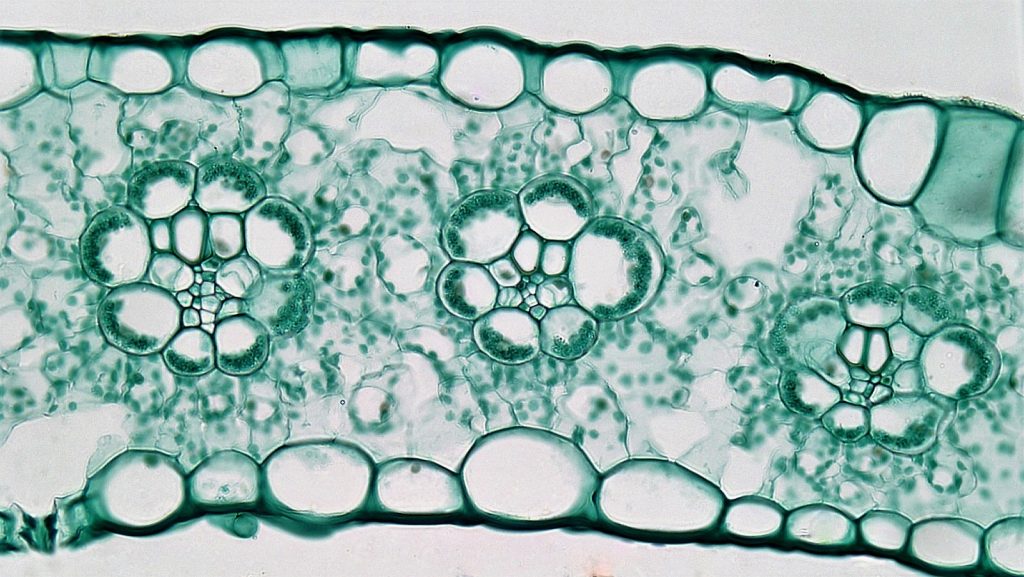
Plants that utilize this dual carboxylation pathway are called ‘C4 plants’ because the first carboxylation produces a four carbon compound as opposed to the pattern in most plants, called C3 plants, where carboxylation produces a three carbon compound. The C4 pathway is outlined below:
- in the mesophyll cells of the leaf a three carbon compound, PEP, is carboxylated by PEP carboxylase, to form a four carbon compound
- the four carbon compound is transported to the bundle sheath cells that surround the vascular bundles (the veins of the leaf)
- the four carbon compound is de-carboxylated, releasing CO2 and pyruvate, a three carbon compound
- the pyruvate is transported back to mesophyll cells where it is converted by into PEP in a process that requires energy in the form of NADPH and ATP
- the CO2 that was released in the bundle sheath is fixed utilizing rubisco in the normal pathway
Note that C4 requires all the machinery and reactions of C3 photosynthesis, it is just that there is an additional set of steps prior to the C3 pattern. C4 photosynthesis is less efficient than C3 photosynthesis because it requires more ATP energy. However, it is more efficient in terms of water use and this is of greater significance in drier regions. Also, for reasons that we won’t go into, C4 photosynthesis is favored at higher temperatures.
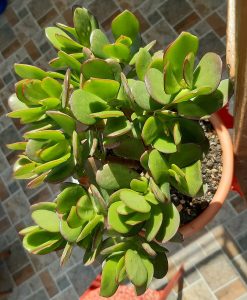
There is a second group of plants that utilize the dual carboxylation pathway but in a modified way. They are called CAM plants. CAM refers to crassulacean acid metabolism because the pattern of behavior shown by this group was first discovered in succulent plants in the Crassulaceae family. CAM plants have several peculiarities: they are usually succulent, i.e., have thick fleshy leaves (Fig. 5), or no leaves and a thick, fleshy stem (e.g. cactus). They typically show a marked daily pattern of tissue acidity with the highest acidity at dawn and decreasing acidity during the daylight hours and increasing acidity during the nighttime. Most peculiar is that they open their stomates at night, not during the day whereas most plants only open their stomates during the day when they can photosynthesize.
Basically, these plants operate just as C4 plants do but instead of having a spatial separation of the two carboxylations as C4 plants have (mesophyll vs. bundle sheath), they have a temporal separation (daytime vs. nighttime). The initial carboxylation occurs at night when stomates are open. The four carbon compound is an acid and causes tissue acidity to increase. During the day the stomates close, carbon dioxide is provided by the decarboxylation of the four carbon acid and tissue acidity declines. The released carbon dioxide is re-fixed via rubisco to form carbohydrates.
Do these plants photosynthesize at night or during the day? It depends on how one might want to define photosynthesis: carbon acquisition is at night but sugar synthesis is during the day. CAM photosynthesis is associated, but not obligately, with succulence. CAM plants are generally found in habitats that are dry, either climatically, e.g., deserts, or because of microhabitat, e.g., epiphytes, plants that are not rooted in the ground but that grow on other plants. E piphytes are often exposed to drought because of their lack of connection to the soil. Cactus and other leafless succulents are commonly CAM plants. CAM photosynthetic rates are very low and CAM plant growth rates are also low. The association of succulence and CAM probably reflects the fact that plants in arid habitats often develop succulence to store water and that succulence is more conducive to CAM because succulent tissues can store more carbon as the four-carbon acid.
Patterns of both CAM and C4 photosynthesis reveal that these pathways have evolved multiple times, i.e., the pathways say little about phylogeny. C4, C3 and CAM are mixed within genera, families and orders. Apparently, it is relatively ‘easy‘ for C4 and CAM to evolve.
Further Reading and Viewing
- “Early Evolution of Photosynthesis” by Robert E. Blankenship. The origins of photosynthesis.
Media Attributions
- Chlorophyll-a-3D-vdW is licensed under a Public Domain license
- NADPH © Tagm2A is licensed under a Public Domain license
- Chloroplast stroma © Somepics is licensed under a CC BY-SA (Attribution ShareAlike) license
- C4 leaf © Berkshire Community College Bioscience Image Library is licensed under a CC0 (Creative Commons Zero) license
- Jade Plant © Sabina Bajracharya is licensed under a CC BY-SA (Attribution ShareAlike) license