Chapter 3: Boundaries
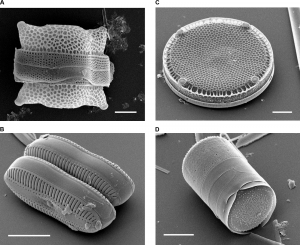
Significance of boundaries
We have defined an organism as living material that is discrete (i.e., bounded) in space and time. Being distinct in space requires a boundary that separates the organism from the ‘outside’. For single-celled organisms the boundary is the outermost component of that cell, and for most of the single celled organisms studied in this course that outermost component is a cell wall, but the chemical and physical nature of the wall varies considerably. For some unicellular organisms there is no cell wall and the outermost layer is a structure called a cell membrane. For multicellular organisms, the boundary is the collective of all the (generally specialized) cells on the organism’s perimeter, its dermal tissues. For most of the organisms studied here the dermal cells have a cell wall and also a specialized coating on the outside. This chapter examines the physical and chemical nature of organism boundaries and also considers the significant functions of the boundary, starting with its influence on something called flux, the movement of materials in and out of the organism.
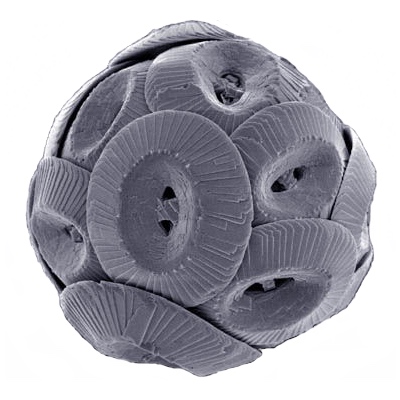
TOPICS
- Boundaries and flux
- Structural, chemical and physical nature of boundaries
- Membrane
- Walls
- Polysaccharide cell walls
- plants, fungi, bacteria, archaebacteria
- Inorganic cell walls
- Internal cell walls
- Polysaccharide cell walls
- Dermal tissue
- Coatings
- Boundaries and structure
- Boundaries and movement
- Boundaries and communication
Boundaries and flux
One reason the boundary is significant because it represents a barrier to movement into and out of the organism. This movement can be described as a ‘flux’ and flux can be modeled as being a function of three parameters present in the following formula (which is actually a model of what causes materials to move):
Flux = (driving force * surface area) / resistance
While in many ways this equation is a simplification, it does point out the three fundamental properties that determine the flow of materials into and out of the cell:
- Driving force—this is generally approximated by the difference in the concentration of something on the inside vs. the outside, but in the case of heat flow the driving force is the difference in temperature; considering the movement of fluids (e.g., air, water) the driving force can be pressure differences. In all these situations, the bigger the driving force, the more flux there is.
- Resistance—the resistance can be described as the difficulty with which materials can move from the outside to the inside, or vice versa. Some materials readily allow the movement of molecules (or heat) through them, i.e., they have a low resistance. Other materials only reluctantly allow materials (or heat) to move through them, i.e., they have a high resistance. It is important to appreciate that resistance depends on the substance/ molecule one is keeping track off; a boundary may have a very high resistance to some molecules and a very low resistance to others. (related terms are conductance and permeability, which, for our purposes, are the reciprocal of resistance)
- Surface area — the more surface area through which materials can flow, the more flux.
As will be discussed in the next chapter, the surface area is determined by the shape and size of the organism. The driving force is determined by where the organism is and what is going on inside and outside of the organism. Organisms can manipulate fluxes by manipulating the driving force. Most of the discussion of these mechanisms is beyond the scope of this book but we will mention some in Chapter 22. In the case of the flow of materials or heat into and out of cells, the resistance to flux is determined by the chemical and physical characteristics of the boundary between the organism and its environment.
Besides being important in influencing the flux of materials into and out of organisms, boundaries serve other important functions that will be discussed after describing different types of boundaries.
Structural, chemical and physical nature of boundaries
Cell membrane
The chemical nature of the cell membrane should be familiar to biology students: a phospholipid bilayer with proteins imbedded in and through it. The two sides of this membrane are ‘w et’ (i.e., hydrophilic) due to the attraction of polar water molecules to the charged phosphate groups of the membrane. The interior of the membrane is dry (i.e., hydrophobic) because lipid molecules carry no charges and have no regions that are even partially charged and to which a polar (= partially charged) water molecule might be attracted. However, there are tubular proteins passing through cell membranes. These tubular proteins may have a ‘wet’ in interior, providing for water filled channels running from the outside to the inside. Some of these channels allow selective passage of ions and many of these can be manipulated (opened or closed). Although the chemical nature of the hydrophobic layer is different in the Archaea, the basic structure of membranes is fundamentally the same for all organisms. Significant to organism function is that the membrane is quite permeable (low resistance) to small, uncharged molecules (significantly O2, CO2 and H2 O) but not very permeable (high resistance) to charged molecules (ions) and to larger molecules, especially if they are not soluble in lipids. The cell membrane generally has little strength and readily yields to internal and external forces, that can cause the cell to change shape. Organisms lacking a cell wall in terrestrial environments cannot be very tall because the force of gravity flattens them. This can be overcome with an internal skeleton (vertebrates) or an external one (exoskeleton), which is a rigid boundary.
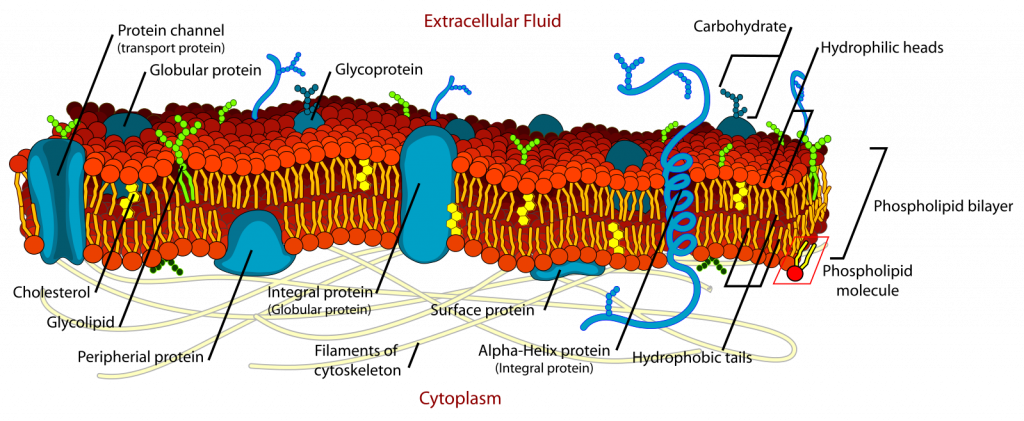
Cell walls
In contrast to the consistent presence and composition of the cell membrane in the cells of organisms, cell walls show much greater variability. Many organisms (e.g., almost all animals) have no cell wall. For the organisms that possess walls, its composition is quite variable (Table 1), although some of its structural features are more consistent.
Polysaccharide cell walls
The cell walls of plants, fungi, water molds, brown algae, red algae and (most) green algae have a similar structure and chemistry. These cell walls are composite structures, and can be considered to be gels. They consist of fibers made of elongate carbohydrate polymers that resist being stretched. These are imbedded in a matrix of different carbohydrate polymers that are highly hygroscopic (water absorbing) (Fig. 4).
Two common fiber materials are cellulose (found in plants, water molds and green algae) and chitin (found in fungi). Cellulose is a polymer made up of glucose monomers, as is the more familiar polysaccharide, starch, but the two have very different roles, one structural and one energy storage. Chitin is also a polysaccharide polymer but the subunits (N-acetylglucosamine) are hexoses with a nitrogen attached (Fig. 5).
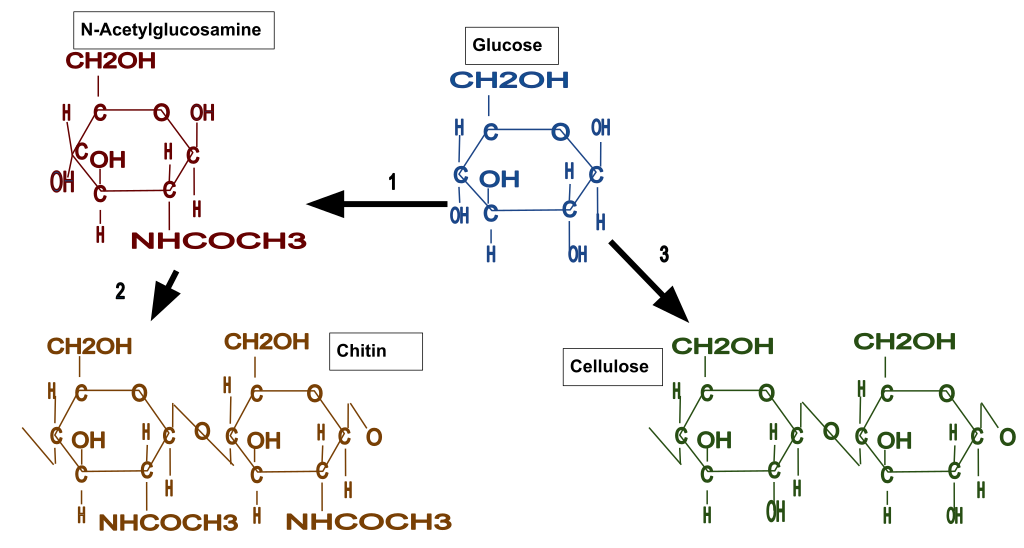
The fibers of cellulose and chitin are consist of multiple (20-100) individual polymer molecules that interact with neighboring molecules to form a crystalline structure of the fiber. These fibers are significant in multiple ways: (1) because there are multiple molecules and they are bound to each other, the fibers, and the wall they are present in, are considerably ‘stronger’ (see below) than would be the case if the individual molecules did not interact to form fibers; (2) the fiber is difficult to digest because the component polymer molecules are not very accessible to enzymes and the fiber itself is not soluble in water, in spite of the fact that the monomers units, e.g. glucose, are very soluble in water.
The fibers of chitin and cellulose are deposited in a matrix of other polysaccharide polymers whose composition is much more variable. This is because the polymer ‘chain’ consists of several subunits, not just one, and also because the linkages between subunits are more variable, not always the same one, and not always ‘end to end’ but with branching (one subunit connected to three other subunits, not just to the ones ‘in front and behind’ it). While these polymers are given names (hemicellulose, pectin, agaropectin), the names refer to groups of chemicals and not to a specific chemical structure and composition. Some of these chemicals bind to cellulose or chitin fibers, connecting them to each other and providing more structure to the cell wall and also allowing the cell walls of adjacent cells to be bound to each other.
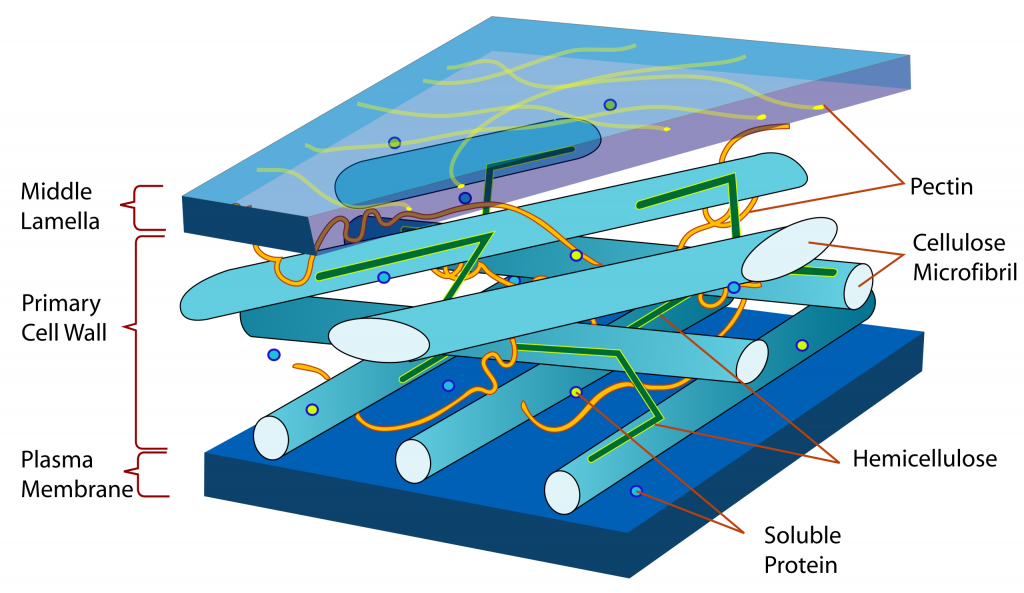
Although they are made primarily of polysaccharides, these cell walls contain some protein molecules. The function(s) of the proteins are not known with certainty. They are probably not particularly significant structurally in the way that the chitin and cellulose are and the way that the intracellular proteins tubulin and actin are. The protein component of the cell wall is probably significant in being able to change, through enzymatic action, the structure of the rest of the wall, e.g., during cellular growth when the wall ‘relaxes’ and allows the internal pressure of the cell to bring about growth (Chapter 25). Wall proteins are probably also significant in allowing material to get through the cell wall (both inward or outward).
Generally, carbohydrate cell walls readily absorb water and can be considered to be a hydro gel. The absorption of water comes from the adhesion of water molecules to the carbohydrate components of the cell wall, in particular the ‘matrix’ polymers (e.g., pectins, hemicellulose) plus the cohesion of water to itself. The hydrophilic nature of the cell wall makes it highly permeable (low resistance) to the movement of water (as a result of either diffusion or as a result of pressure differences, i.e., mass flow (m ore discussion on this when discussing material movement, Chapter 24). Small water-soluble molecules also move readily through the wall both as a result of their individual diffusion and as a result of being dissolved in water that may be moving via mass flow. However, dissolved ions may be slowed by becoming bound to charged components of the polysaccharides.
Under some circumstances, the cell wall of plants can shift from being hydrophilic to being hydrophobic as the result of the deposition in the cell wall of ‘water-proofing’ materials called cutin and suberin. These materials are found in cell walls of certain plant tissues and their ability to retard the movement of water is significant. Both suberin and cutin are mixtures of multiple compounds including polymers with hydrophobic subunits and wax molecules. Like the lipid portion the cell membrane, these chemicals are ‘dry’ (hydrophobic) and water movement through walls with these materials is much retarded. While there are some chemical differences between the two, the primary distinction between cutin and suberin is location. Cutin is deposited in a coating called cuticle, present on the external walls of cells located on the outside of the aerial portions of plants. Suberin is deposited in the walls of certain cells as they are produced. Suberin is found in the above-ground portion of the plant in places where growth or mechanical damage has eliminated the cuticle. Suberin is also found in the below-ground portion of most vascular plants in a cylindrical tissue called the endodermis, whose significance will be discussed later.
A final component found in some polysaccharide cell walls is lignin, a material absent in non-vascular plants (e.g., mosses) but found in the cell walls of most vascular plants, and a material that is highly significant to their evolutionary success, i.e., their prevalence in today’s flora. Structurally, lignin is similar to pectins and hemicellulose, i.e., a matrix that surrounds cellulose fibrils. Lignin is produced in what is described as the secondary cell wall, a component of the cell wall present only in certain cells. The secondary cell wall is laid down after cells have stopped growing, necessarily so because lignin is a rigid material that will not yield readily, therefore preventing the cellular expansion needed for growth. Lignin is the material that makes wood tissues woody. It is stiff and rigid and allows plants to be tall by resisting the compressive force of gravity. Like hemicellulose and pectin, lignin is a complex polymer with subunits that are not all the same and are not always connected the same way. Moreover, the subunits of lignin are not sugars, they are phenolic molecules chemically quite different from sugars. Lignin polymers extend in three dimensions, forming a solid material. Lignin is hard to digest and its breakdown products are phenols, chemicals that are poisonous to many organisms. Hence, lignin is resistant to degradation. The secondary cell wall is deposited inside of the primary cell wall and it necessarily shrinks the space available to the membrane bounded cytosol, sometimes eliminating it almost completely. Most cells with secondary cell walls are short-lived and are structural, providing function to the plant when they are dead.
Bacterial cell walls
Not all bacteria possess cell walls but the ones that do possess a wall material unique to bacteria called peptidoglycan. As the name implies, peptidoglycan has components that are peptides (sequences of amino acids) and components that are sugars (carbohydrates). Unlike the polysaccharide wall materials described above that are chains of monomers, peptidoglycan polymers form a mesh, a three-dimensional molecule (like lignin) with units linked not just end-to-end but also above-and-below and side-to-side. Also significant is the fact that many bacteria, including ‘gram-negative’ bacteria and cyanobacteria, have an ‘outer membrane’, a second phospholipid membrane that occurs outside of a thin peptidoglycan cell wall. Some bacteria possess one additional layer, an ‘S-layer’, a protein layer on the very outside of the cell, outside the outer membrane of gram-negative bacteria and the peptidoglycan cell wall layer of gram-positive bacteria.
Archaea cell walls
Recall that Archaea and Bacteria are prokaryotes and used to be considered a single group. One of the several features that distinguish Archaea is a distinct cell wall structure one that lacks peptidoglycan. The chemical composition of Archaeal cell walls is diverse and some have a cell wall composed of a carbohydrate/peptide material similar to peptidoglycan. Like some Bacteria, most Archaea are bounded on the outside by an S-layer, a self-assembling structure composed of globular proteins or glycoproteins.
‘Inorganic’ cell walls’
All of the cell wall materials discussed so far would be considered ‘organic’, a flexible term whose exact meaning wanders considerably. In this context it refers to ‘biological materials’, molecules found in living things, as opposed to inorganic molecules, those generally found in non-living things, e.g. rocks/minerals. Two important cell wall materials, calcium carbonate and silica dioxide, are most commonly found as minerals in rocks. However, these minerals are in some sense ‘organic’ because they can be produced by biological processes, cells produce conditions whereby calcium carbonate or silicon dioxide is precipitated out of solutions. In fact, their presence in some rocks is completely due to their manufacture by marine organisms whose remains became deposits at the bottoms of oceans and eventually in rocks. The ‘White Cliffs of Dover’ (Fig. 6) are formed from a massive deposit of coccolithophores, a type of marine algae that produces distinctive looking calcium carbonate plates as its outside boundary (Fig. 2). Although not as common or extensive as calcium carbonate cliffs, there are similar deposits of ‘diatomite’, a sedimentary rock formed from deposits of diatoms, another unicellular photosynthetic organism that produces an external skeleton, i.e., a cell wall, made of silica dioxide (Fig. 1). Exactly how the material is precipitated in such a precise, and often ornate, manner is not known with certainty.
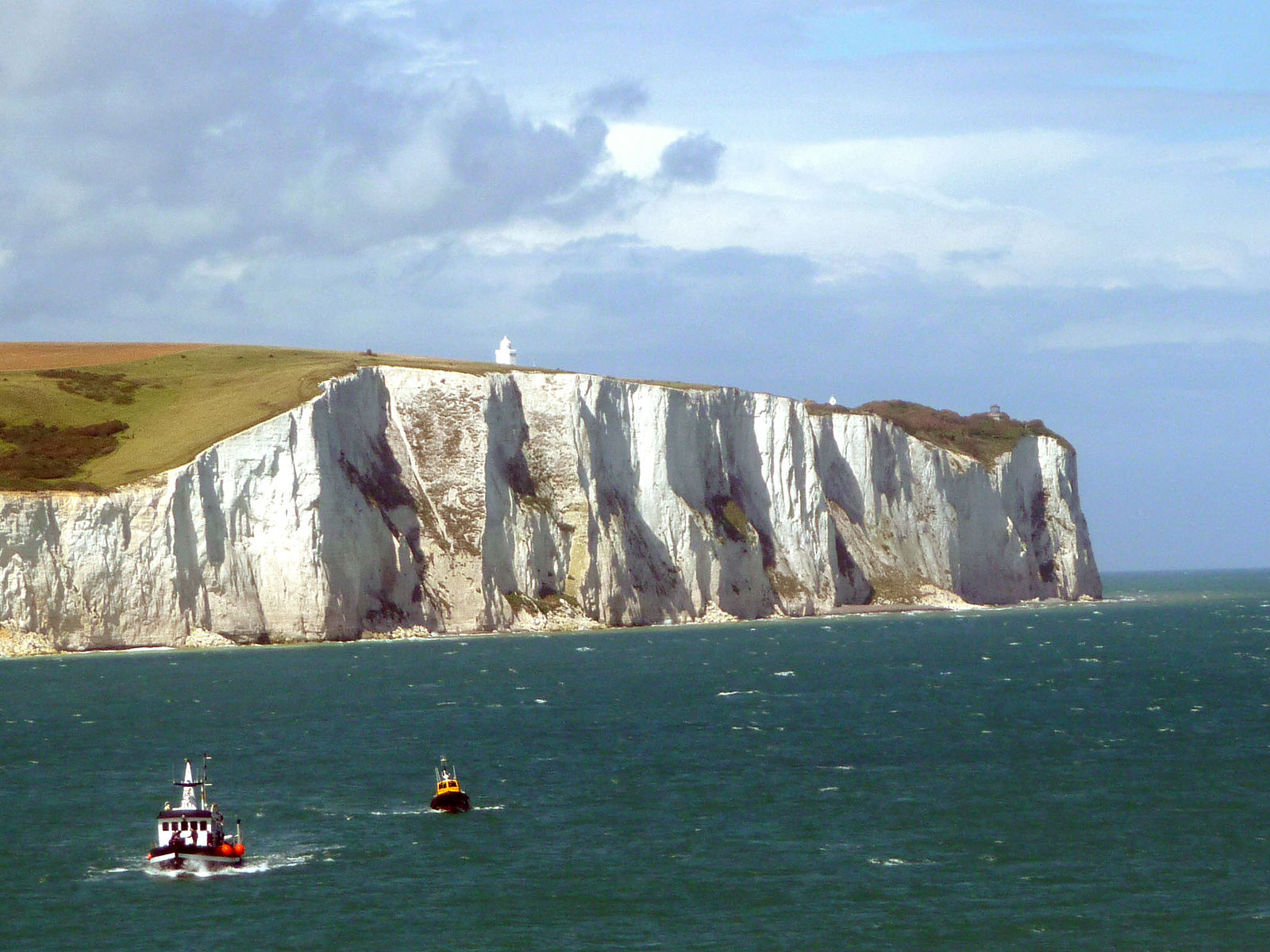
‘Internal’ cell walls
A few organisms have an unusual structure where wall-like materials (i.e., structural components) occur just inside the membrane. One example is gram negative bacteria where there is a wall inside a membrane, but these bacteria also have a second membrane in its normal location, inside the cell wall. In contrast, dinoflagellates have plates of cellulose enclosed in membranes that occur inside of the plasma membrane. Cryptomonads have wall-like glycoprotein plates both inside and outside of the plasma membrane, forming a structure called the periplast that is unique to this group of organisms.
Dermal tissues of multicellular organisms
Most multicellular organisms have groups of cells, called dermal tissues, that form a ‘skin’ to an organism. These cells / tissues will be considered anatomically in a later chapter but they generally are cells tightly bound to each other (no spaces between them) so that they collectively form a boundary to the organism. Often these cells have different components in their cell wall (e.g., cutin, suberin, lignin) and/or produce a secretion on the outside (cuticle, see below) that is important to their functioning. Additionally, many multicellular organisms produce structures, organs, that have specialized boundaries that are significant to their role. In inanimate life the most common of these are organs associated with reproduction, e.g., spore cases (sporangia), fruits and seeds. The ‘skin’ of these structures may be important in protecting the structures inside, e.g., the sporangium of mosses, the outside of an acorn (a fruit wall), the seed coat of apple seeds (in the latter two cases it is lignin depositions in the cell walls of dermal cells that are particularly important). The ‘skin’ of reproductive structures must eventually open up and allow the dispersal/release of its contents and sometimes features of the ‘skin’ actively participate in dispersing its contents (see discussion below on ‘explosive’ movements). In other situations, the permeability of the coating, in particular how much water/oxygen enters, can significantly influence the behavior of the enclosed structures.
Specialized coatings
Some organisms /colonies of organisms cover themselves or part of themselves with some sort of coating. A number of algae and bacteria coat themselves in a polysaccharide gel outside of the cell wall. A striking example of this is with some species of Nostoc, a colonial, filamentous cyanobacterium that sometimes forms gelatinous sheets or spheres that may be several centimeters across (Fig. 7). The bulk of the sphere is a polysaccharide secretion deposited outside the cell walls of individual cells. Coatings may be significant to the organism for a variety of reasons including: retention of water, protection, adhesion to substrates, keeping a colony of cells together, buoyancy. Similar coatings are sometimes important in producing biofilms, communities of one to several organisms including bacteria, archaea, fungi and others, that coat surfaces (e.g., dental plaque) and are sometimes important ecologically and to human disease).
Fig. 7 Nostoc, a cyanobacterium, can form spherical structures up to several centimeters in extent (left photo), the result of polysaccharide secretions. The photo on the right shows the filaments of the algae, the spaces in between the filaments are filled with polysaccharide hydrogel secretions.
Cuticle
Most plants, who are terrestrial organisms exposed to a drying atmosphere, have a coating on the outside called a cuticle (Figure 8) that lessens water loss. The cuticle is a complex mix of chemicals including cutin (mentioned above as a component of some cell walls) and other similar (hydrophobic) constituents. The cuticle is much more impermeable to water (it has a high resistance to water movement) than the membrane because it is substantially thicker and also because proteins do not span across it. The cuticle’s high resistance to water movement is significant because it lessens water loss from plants to the atmosphere. However, the cuticle is also impermeable to gases, in particular carbon dioxide, and this feature has important consequences for photosynthesizing plants. The cuticle is produced by the cells on the outside of the plant, and the outer part of the cell walls of these cells have extensive deposits of cutin. In addition, hydrophobic materials are deposited completely outs ide of the cell wall (outside the area where cellulose microfibrils are present). The mechanism whereby materials can be deposited outside of the cell walls is not completely understood.
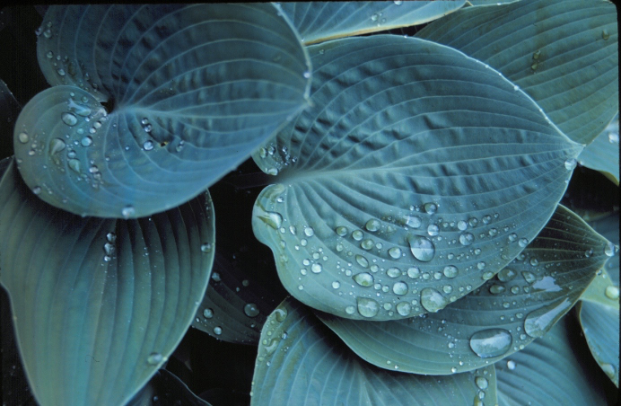
In addition to reducing water loss, the cuticle is a physical barrier to the entrance of organisms into plants, and it also serves to absorb and reflect UV radiation, thereby protecting tissues within. Also, the dryness of the cuticle, along with other specific chemical features, makes it a very inhospitable place for the growth of other organisms, adding to its protective function. The cuticle of many plants may be ruptured by growth from within (discussed later). In these cases, the protection of the cuticle is replaced by a new layer or layers of cells that are produced with suberin in their cell walls, forming the outside portion of what we know as bark.
Spropollenin
Pollen grains (of seed plants) and the spores of a variety of organisms are coated with sporopollenin, a chemical that is particularly resistant to degradation and is thought to be significant in protecting against desiccation, oxidation and enzymatic degradation. It is the resistance of sporopollenin to breakdown that allows pollen buried several hundred million years ago in sediments to be preserved and recognized. Its exact chemical nature has not been determined (partly because it is so hard to breakdown!) but it has some similarities to cutin in having hydrocarbon components as well as phenolic components.
Boundary diversity
The following table summarizes boundary materials for organisms that we cover (plus a few others) grouped grouped by whether they are unicellular or multicellular and whether or not they possess a cell wall.
Boundary and organism type | Organisms | Features | |
Unicellular organisms with no walls | Some bacterial groups | Mostly parasites | |
Cellular slime molds | For parts of their life cycle they do have walls | ||
Plasmodial slime molds | Like a giant amoeba | ||
Euglenoids | No wall, but the membrane is reinforced with protein filaments forming a structure called a pellicle, often exhibiting parallel striations on the outside | ||
Cryptomonads | Wall-like materials (glycoproteins) both inside and outside of the plasma membrane, forming a structure called a periplast. | ||
Dinoflagellates | Often possess multiple ‘plates’ of cellulose that lie interior to the cell membrane | ||
Multicellular organisms with cells with no walls | Animals | Many do have coatings (‘skin’) that are groups of cells | |
Unicellular organisms with cell walls of some sort | Bacteria (most), including all Cyanobacteria | Wall contains a peptidoglycan polymer with polysaccharide and amino acid components. Some bacteria have a second, ‘outer membrane’ outside the peptidoglycan layer | |
Archaea | Wall contains a polymer similar to peptidoglycan with polysaccharide and amino acid components | ||
Diatoms | Wall is made of silica (SiO2) — Silicon (Si) is an element that most organisms do not accumulate. The wall is also distinctive because it is not organic (i.e. carbon based), does not absorb water and is very rigid | ||
(some) Green algae (Chlorophyta) | A variety of wall materials (and sometimes none at all) many green algae have walls containing cellulose, a polymer of glucose | ||
Haptophytes (Coccolithophores) | Wall consists of round plates of calcium carbonate outside the cell membrane | ||
Bread molds (Zygomycota) | Cell walls are composed of chitin, a polymer of acetyl glucosamine units (essentially sugar units with a nitrogen attached). The same material is found in the exoskeleton of insects and mollusks. | ||
Water molds (Oomycota) | Cell walls are contains cellulose, a polymer of glucose units. | ||
Multicellular organisms with cell walls | Club fungi (Basidiomycota) | As is the case in all fungi and in the bread molds, the cell wall is composed of chitin, a polymer of acetyl glucosamine units (essentially sugar units with a nitrogen attached). | |
Sac fungi (Ascomycota) | Same as Club fungi | ||
Red algae (Rhodophyta) | Wall contains cellulose and sulfated polysaccharides. | ||
Brown algae (Phaeophyta) | Wall has small amounts of cellulose with large amounts of alginate, a polysaccharide polymer composed of uronic (sugar-acid) units | ||
Green algae (Chlorophyta) | Wall varies but some with cellulose as the main constituent; other green algae have mannans (polymers of mannose), xylans (polymers of xylose), glycoprotein polymers and some have no wall at all | ||
Plants (mosses, conifers, flowering plants) | Cell wall contains filaments of crystalline cellulose connected by hemicellulose polymers and imbedded in pectin polymers. Many plant cells develop an inner secondary cell wall containing cellulose and lignin, a complex, phenolic polymer. |
Cell walls, cell membranes and structural integrity
One reason that an organism’s boundary is significant is because the boundary resists physical forces that are acting on the organism. These forces can come from the outside (e.g., wind, gravity) or from the inside (internal pressure). For unicellular organisms it is the strength of the boundary that prevents (or allows) deformation that would come about as a result of these forces. For multicellular organisms it is the boundaries of individual cells and also their linkages to each other that determine how the organism will respond to external forces.
Osmotic forces
A common factor that might cause a cell to change shape is ‘osmotic disruption’, brought about by osmosis, the diffusion of water. All other things being equal (more in Chapter 22) water moves by diffusion from areas where it is purer (i.e. has less solutes) to areas where it is less pure (has more solutes). Since living things acquire and manufacture solutes and the impermeability of the cell membrane allows these solutes to be concentrated inside cells, cells are often are in situations where water is going to spontaneously move into them. The cell membrane offers little resistance to expansion and is not able to be stretched. Consequently cells/organisms with only a membrane will burst if exposed to pure water, or any water that is purer than that inside the organism, unless they have mechanisms to eliminate the water. The presence of a cell wall outside the membrane solves this problem because it resists expansion. This allows the cell to pressurize, and this pressure prevents the entry of more water (note that diffusion is NOT just dependent upon differences in purity, as it is often described. It is also dependent upon pressure, more in Chapter 24). It is important to note that the strength required to resist expansion is ‘tensile’ strength. The cell ‘resists’ water absorption because components of the wall (e.g., cellulose or chitin microfibrils) are resistant to being stretched.
If the cell with a cell wall were in an environment causing it to lose water, e.g., an aquatic habitat high in solutes or in a terrestrial habitat where organisms are losing water to a drying atmosphere, water would diffuse outward and the presence of a wall would not prevent the cytosol from collapsing unless the membrane were somehow glued to the cell wall to prevent it. This is not thought to be the case. Moreover, for plant cells with only primary cell walls (no lignin reinforcement) or fungal cells, the wall is not strongly resistant to compression and if water leaves the cell the cell will shrink in size (collapse). Note that although cellulose fibers have a high tensile strength, they are not very resistant to compression, i.e., have little compressive strength. Consider a string (which often times is actually a collection of cellulose microfibrils): you can pull on the string and it resists stretching but it is very easy to collapse the string, it has very little compressive strength.
Walls and ‘structural integrity’
Cells and organisms do need compressive strength to: (1) provide protection from certain predators who would like to crack them open to get to the goodies inside, and (2) allow the organism/cell to resist various forces in the environment, e.g., gravity. Resisting forces becomes more significant if organisms are bigger, especially for organisms in terrestrial environments where the surrounding medium (air) provides little support. In terrestrial environments, organisms will be collapsed by gravity if they are more than a few centimeters in height unless they have structural strength to resist it. Three-dimensional wall materials (e.g., peptidoglycan, calcium carbonate, silica dioxide), can resist compression but these wall materials are only present in very small organisms. Plants are ‘big’ and terrestrial, how do they resist gravity? Walls with lignin, another 3-dimensional material, will resist compression, but not all plant cells have lignin. Plant cells lacking lignin have structural integrity against gravity because of the combination of water, a membrane that ‘holds’ solutes but allows water movement, and a cell wall that has tensile strength. Water is very difficult to compress as long as it is contained in something that doesn’t allow water to escape. It might seem that water should be able to be ‘squeezed out’ of cells by the force of gravity but this doesn’t happen because water movement out, as a cell is squeezed and pressurized by gravity, is balanced by water movement in due to purity (the low water purity inside is because of solutes, Chapter 24). To summarize: plant cells without lignin resist the force of gravity because is incompressible (high compressive strength) and is located within a selectively permeable membrane surrounded by a cell wall with tensile strength.
This structural feature is demonstrated in wilting plants. Deprived of a supply of water, many plants are unable to resist the force of gravity and to maintain their structure. The cells, mostly with only primary cell walls (no lignin) in and of themselves cannot resist compression, they need water, and if re-watered a wilted plant will once again stand up against gravity Fig. 9). Woody plants, at least the woody parts of woody plants as well as some herbaceous plants do not wilt because they have cells with secondary walls containing lignin that provides the compressive strength to resist the forces of gravity.
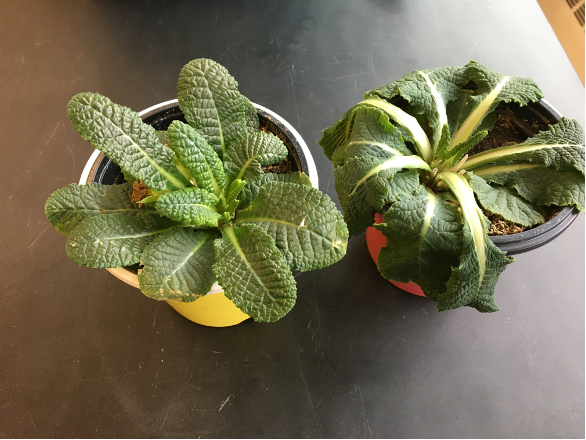
Although most cell walls resist expansion, the resistance is not absolute and cells can yield (i.e., expand) to some extent when pressures increase inside them. The yielding of cell wall is both elastic (the wall yields, but returns to its original form when the force, internal pressure, is eliminated) and also plastic (the wall yields but does not return to its original shape if the internal pressure is reduced). Because cell walls are present even in newly created cells, plastic deformation is essential for cellular growth.
Considering a tree trunk being pushed by the wind, the windward side needs tensile strength, the lee side needs compressive strength. Woody stems are almost entirely composed of lignified cells that have both tensile strength from the cellulose and compressive strength from the lignin. Such a composite material is comparable to ‘reinforced’ concrete (concrete poured around wire/steel rods) or fiberglass (resin poured around glass fibers), where the matrix of concrete/resin provides compressive strength and the fibers of wire/glass provides the tensile strength. Moreover, the lignin in plant cells was deposited while the cellulose was under tension, because the cell was pressurized when the lignin was deposited in the secondary cell wall. This produces a composite material like pre-stressed concrete, a concrete made by pouring concrete around steel cables that are under tension. Pre-stressed concrete has superior strength when compared to regular reinforced concrete. Finally, a tree trunk blown by the wind not only has to have structurally strong cells, the cells need also to stick to each other because separations between cells will cause structural failure, and indeed when stems break or lumber fails it is both because of separations between individual cells and also failures within individual cells.
Boundaries and organism movement
Organisms move by manipulating their boundaries relative to the environment. For familiar organisms (lions and tigers and bears) this is done with appendages (legs, fins, tentacles) whose positions are manipulated internally. This is comparable to the movement brought about by flagellae and cilia, which are extensions of the boundary of cells that often provide motility to unicellular organisms, including most of the groups covered here: most archaea (e.g. Halobacterium), most bacteria (e.g. Agrobacterium, Rhizobium), euglenoids (e.g. Euglena), dinoflagellates (e.g. Gonyaulax), cryptomonads, some of the green algae (e.g. Chlamydomonas). Flagellae and flagellar motility is commonly found in the reproductive cells (e.g. sperm, zoospores) of most multicellular organisms, and, as considered in Chapter 2, these cells might be considered organisms. Thus flagellated mobility is near ubiquitous, lacking only in a few groups, all of them ‘inanimate’, being absent in all red algae, all flowering plants (and almost all seed plants), almost all fungi (except in some Chytrids), and almost all diatoms.
But there are other means of movement and the diversity of organisms that we cover illustrate a diversity of movement mechanisms, all of which, like flagellar movement, are related to manipulation of boundaries. Some examples are given below:
Flagellar motility
Cytoplasmic streaming
Like amoebae, the amoeboid cells of cellular slime molds move by pushing and pulling on their boundary by means of microtubules, keeping the volume of the beast constant while changing its form. This allows them to move through their environment or to flow around a particular food item. Plasmodial slime molds, though much bigger and multi nucleate, are able to move in a similar manner. Their cytosol is seen to stream back and forth but more so in one direction, propelling the organism in the direction of greater flow.
Euglena motion
Organisms in the Euglena group can move by both flagellar movement and by a movement that involves reshaping their form, comparable to an amoeba or slime mold.
Growth
Fungi and plants move by growing, which requires the extension of boundaries into new territory. This process will be covered later but it requires the extension of individual cells, accomplished by the pressure inside these cells exceeding the tensile strength of the cell wall and also exceeding the resistance to movement provided by the medium that they are moving through (e.g., soil).
Stomatal movement
Vascular plants and a few non-vascular plants have the ability to change the shape of pairs of cells (guard cells) in the skin tissue of (generally) leaves, thereby opening pores (stomata) in the leaf surface. This is accomplished by means of changing hydration levels of cells, thereby changing their pressure and shape and in turn opening a hole in between them. Stomatal movement ties into two topics of this chapter: (1) the change in shape in guard cells is a consequence of asymmetric deposition cellulose microfibrils in the cell walls, (2) the opening of stomates dramatically changes the dermal permeability to carbon dioxide and water, allowing water to escape the leaf and carbon dioxide to enter.
Leaflet/organ movement
In addition to moving by growing, a number of plants are able to move appendages, usually leaves. This is accomplished in a manner similar to the operation of stomates: by changing hydration levels of specific cells and groups of cells, thereby changing their pressure and volume and in turn changing their shape. In flowering plants, the changes in cellular shape happen in special cells at pivot points that allow leaves to move. In mosses, changes in cellular shape happen in all the cells of the ‘leaves’ (microphylls) , sometimes causing them to twist and curl up next to the main axis of the moss. Most mosses also possess appendages called peristome teeth at the end of the spore-producing capsule. Changes in the hydration of the cells in these teeth change their shape, which allows the capsule to close in times of high humidity, when spores are less likely to be dispersed, and open in times of low humidity, when spores are more likely to be dispersed. Although not a boundary movement, a similar mechanism and motion happens with groups of cells called elaters that are present in the sporangia (containers where spores are produced) of horsetails and liverworts and whose movement aids in the dispersal of spores.
‘Explosive’ movements
Mentioned earlier was the fact that as cells absorb water and expand, the change in size may be elastic, in which case energy is being stored in the wall; this energy can be utilized to cause movement. Such an energy storage and a resultant movement is significant in spore and seed dispersal. A container, which may be a cell (e.g. the basidium of club fungi (Basidiomycota) or may be a container with a wall of dermal cells (the fruit of dwarf mistletoe or squirting cucumber, both flowering plants) becomes pressurized and stores energy in the wall(s). Then a part of the wall(s) breaks open and the pressure is rapidly released, expelling the contents (spores or seeds). A similar phenomenon occurs in Pilobolus, a bread mold (Zygomycota) except that what is expelled is the whole spore container (sporangium), occasionally with other organisms hitching a ride. Dehydration can also result in movement. Cells in the fruit wall of some fruits (e.g., witch-hazel) and also in the spore containers (sporangia) of many ferns dehydrate as the fruits/sporangia mature. Dehydration results in shrinkage that is resisted by the cohesive strength of water bound to cell wall materials. More and more force builds up as more and more water is lost. Eventually the force exceeds the cohesive strength of the water in the walls of the cells, again causing the structure to rupture. This is often accompanied with an extremely rapid change in the form of the fruit/ spore capsule that can result in the forceful release of seeds/ spores, like a catapult.
Boundaries and communication/sensation
A final point about boundaries is that they play a role in the sensation of the environment and communication between organisms. Organisms need to sense their environment, which means that either signals need to get through the boundary or alternatively that there exists a sensor molecule that extends through the boundary and allows a molecular interaction outside to cause a response inside. Both of these things happen. It is also the case that important signals are actively transported out of organisms and that some signals are components of the boundaries (e.g. cell wall compounds, or derivatives of enzymatic action on cell wall compounds). A great example of this is the communication between Rhizobium, a nitrogen fixing bacteria and the plants they associate with.
Further Reading and Viewing
- “Squirting Cucumber: The Plant That Explodes” by Animalogic. Exploding fruits.
- “Exploding Cucumbers! | Slo Mo #36 | Earth Unplugged” by BBC Earth Unplugged
- “Exploding myths about seed dispersal” by Stuart Gillespie
- “See the Plant Kingdom’s Hidden Microscopic Wonders” by Michael Greshko. Nice images of plants, see in particular the first image showing the cuticle coating on part of a moss and images 3 and 11 that show the interlocking nature of a plant epidermis (“skin”) cells as well as the stomates. And images 17 and 18 show the “glass houses” of diatoms.
- “The Formation and Function of Plant Cuticles” by Trevor H. Yeats, Jocelyn K.C. Rose. Good technical discussion of the plant cuticle.
- General Microbiology by Linda Bruslind. Good discussion on the membranes of archaea.
Media Attributions
- Diatoms © Mary Ann Tiffany, San Diego State University is licensed under a CC BY (Attribution) license
- Coccolithus pelagicus © Richard Lampitt, Jeremy Young, The Natural History Museum, London is licensed under a CC BY (Attribution) license
- Cell membrane detailed diagram © LadyofHats Mariana Ruiz is licensed under a Public Domain license
- Chitin, glucose, and cellulose © Arion422 is licensed under a CC BY-SA (Attribution ShareAlike) license
- Plant cell wall diagram © LadyofHats is licensed under a Public Domain license
- White Cliffs of Dover © Immanuel Giel is licensed under a CC BY-SA (Attribution ShareAlike) license