Chapter 24: Material movement and diffusion’s multiple roles in plant biology
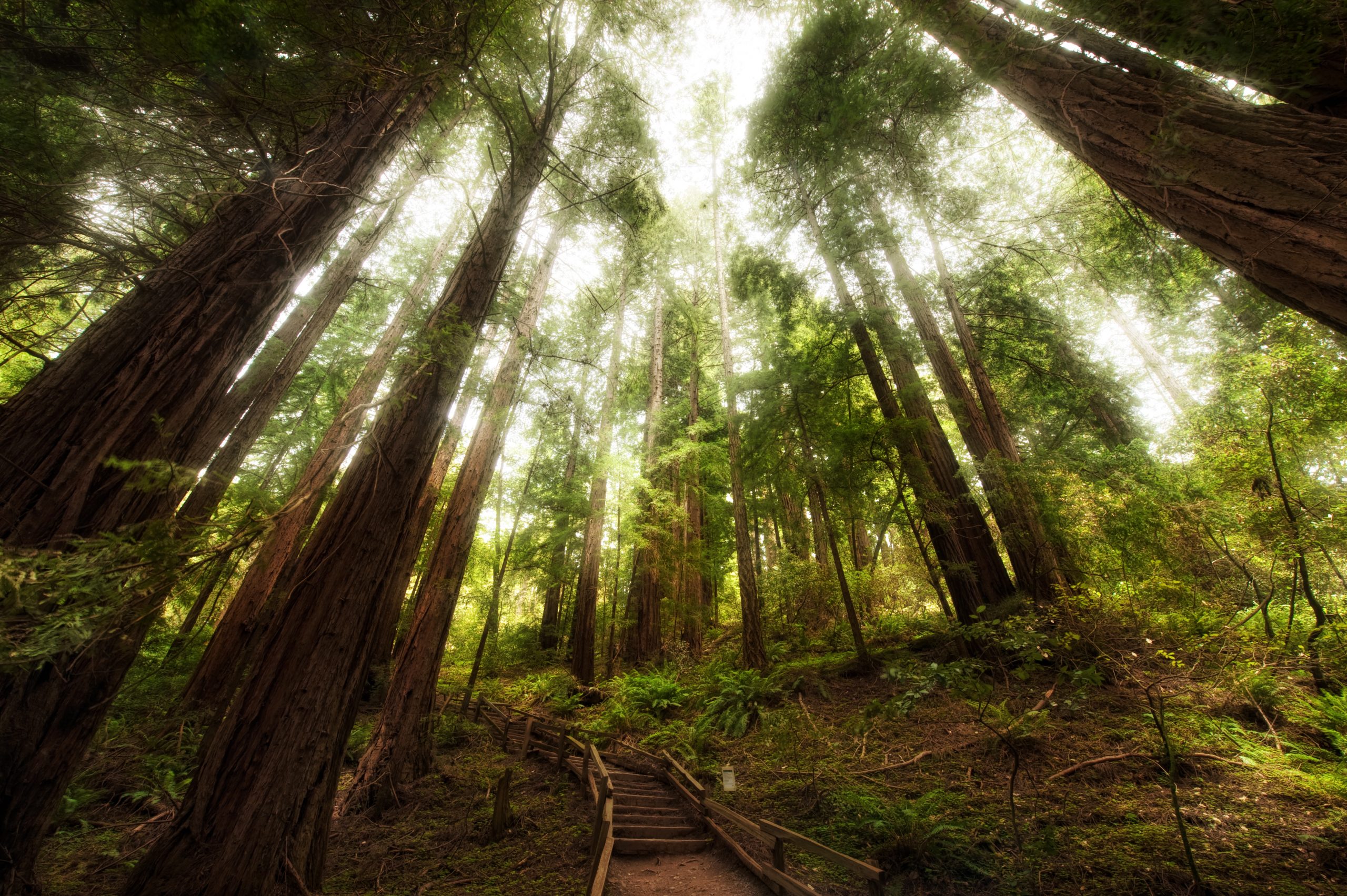
In addition to needing materials, organisms need to move materials: materials need to be moved from where they are acquired or synthesized to other places where they are utilized; materials may need to be transported to storage sites and also retrieved from storage sites; chemical signals may need to move from a place of sensation to a place of response.
Materials are moved in three basic ways in organisms, two of which occur in non-living systems as well: (1) materials move by diffusion, which is a consequence of the fact that all molecules at a temperature above absolute zero (i.e., all molecules!) are moving in a random thermal way; (2) fluid materials (i.e., gases and liquids) move by mass flow, from high pressure to low as long as there is an open pathway that allows the fluid to move; (3) materials move as the result of forces developed as a consequence of chemical interactions unique to biological entities, for example the rotary motors of flagella that create a rotational movement that ‘runs on’ proton movement down an electrochemical gradient and the so-called motor proteins, which can use chemical energy (generally supplied by ATP) to do physical work (i.e., pushing or pulling a molecule, applying force over a distance) as in a muscle. Note that motor proteins are highly significant in multiple ways, not just in muscles. They can divide cells, move chromosomes and produce cytoplasmic streaming, a process that is significant to larger cells because diffusion is ineffective except over very short distances. The larger cells found in some of the algae, and especially in the coenocytic/siphonaceous cells (see Chapter 4) are highly dependent on the ability of motor proteins to transport material within the cell. Of particular importance to plants is the ability of a large, multicellular organism to move materials throughout the organism, a process that is accomplished by a combination of diffusion and pressure flow.
TOPICS
- Diffusion
- A simple but insufficient model
- Osmosis — the diffusion of solvents, including water
- Effects of pressure on diffusion
- Combining the effects of purity and pressure
- Plants and Fungi use manipulations of pressure and osmosis multiple ways
- Structurally
- Growth
- Guard cell movements
- Leaflet movements
- Long-distance transport
- In the phloem
- In the xylem
- the patterns
- the mechanisms
- the problem of cavitation and trade-offs in xylem anatomy
- rare cases of pressurized xylem
Diffusion
A simple but insufficient model
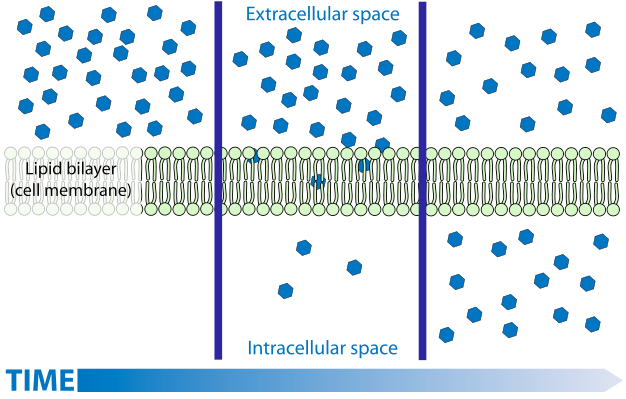
Diffusion is a process familiar to most. While the diffusion of gases and solutes is easily understood by simplistic models, the understanding of the diffusion of liquids, in particular water, is much more challenging and is often muddled by the imprecise application of terminology. The diffusion of gases and solutes is described as a spontaneous movement from regions of higher concentration to regions of lower concentration. The explanation for this spontaneous process is easily linked to kinetic theory—molecules move in a random way because of thermal energy. As a consequence of this random movement, there is a net flow of matter from places where there are more molecules (i.e., higher concentrations) to regions where there are fewer molecules (lower concentrations). If dealing with mixtures (a gas with more than one component), each component will move independently of the others.
Liquid water diffusion (osmosis) is NOT always from ‘high concentration to low ’
However, this model (explanation) is not readily applied to liquid water with solutes, or to solutions in general, largely because the idea of ‘concentration’ varies. Concentration is not as precise a term as one might think, it can be expressed in several different ways (mass concentration, number concentration, molarity, molality, mole fraction). In the simplistic model of diffusion, the most appropriate measure would appear to be (number) concentration (number of molecules per unit volume) since random movement would move molecules from where they have more molecules per unit volume to where they have fewer molecules per unit volume. However, when considering the solvent (not the solute), the number concentration is not an accurate predictor of diffusion. For water and for most solvents the number concentration of the solvent changes very little as solutes are added, yet adding solutes can have a substantial influence on the diffusion of solvent. And while water volume changes little as solutes are added, the extent that it does change varies with different solutes. But the effect on the diffusion of water is not controlled by the specific solute added but only by how much solute (number of particles) was added. Consider that while the addition of most solutes causes the water to slightly increase in volume (the number concentration goes down — the same number of water molecules are now in a larger volume), the addition of some solutes can cause water to contract (i.e., the number concentration goes up — the same number of water molecules are now in a smaller volume). If the (number) concentration of water is what directs its movement one would expect different solutes to have different effects on the diffusion of liquid, depending upon how much they caused the solution to change volume (and number concentration). And you actually would expect that some solutions (the ones that cause water to shrink) would have water diffuse from the solution into pure water. This never happens! Keeping all other factors constant, water always diffuses from where it is pure into any solution, regardless of the solute. And, at low concentrations, there is little to no effect of the particular solute — they all have the same effect on diffusion regardless of their impact on water’s number concentration. It is the concentration of the solute NOT the concentration of water molecules that is directing diffusion: all other factors being held constant, water always diffuses from where there is a lower solute concentration to where there is a higher solute concentration. From this, one can conclude that it is the purity of water, not its concentration, that drives diffusion. This may seem like a subtle difference but it actually reflects some very profound features related to the laws of thermodynamics. Purity relates to entropy, and entropy is known to ‘drive’ spontaneous processes. Moreover, our ‘mental image’, i.e., model, of what causes diffusion, doesn’t work — solvent molecules do NOT go from where there are more of them to where there are less of them, they tend to go from where they are purer to where they are less pure.
The effect of pressure on diffusion
A second key reason that the description/model that describes diffusion as occurring from ‘high concentration to low’ is deficient is that it fails to consider the effects of pressure. Pressure is the most familiar reason that fluids move (wind, water flow in pipes, blood flow in animals) but these movements are not diffusion, they are something called ‘mass flow’, a movement that depends only on pressure differences, and a movement that will occur whenever there are pressure differences and an open path for fluid flow. But when mass flow is impossible (because there is no ‘open path’) pressure can also influence diffusional movement: water will diffuse from areas of high pressure to areas of low pressure. This is especially important for cells possessing both a cell membrane and a cell wall. While the membrane allows there to be different purities of the solvent (water) inside vs. outside the cell, the wall allows there to be different pressures inside vs. outside the cell, and both purity and pressure are important in dictating the diffusion of water.
Combining the effects of purity and pressure
All other things being equal, water moves by diffusion from regions of higher pressure to regions of lower pressure and also from regions of high purity to regions of low purity. These two factors can ‘balance’ each other and it is possible to have NO diffusion between an area of low purity and high pressure connected to an area of low pressure and high purity. As an example, if you have water of low purity confined in a rigid container (i.e., a cell with a cell wall) and it is put into pure water, water will move into the cell, increasing the pressure in the cell. Eventually, a pressure will be reached where there is no more diffusion into the cell. At this point, the pressure differences between the inside and outside are matching the purity differences between the inside and outside.
Unfortunately, there is no easily conceptualized model for the diffusion of liquid water as there is for the diffusion of gases and solutes. A rigorous model of the diffusion of liquid water requires the application of concepts from a thermodynamic parameter called water potential. The basic idea is relatively simple: osmosis (the diffusion of liquid water) is a spontaneous process and any spontaneous process must result in a decrease in the amount of energy available to do work (the ‘free energy’). Generally, two key factors affect the free energy of water (its water potential): the pressure (which increases its water potential) and the presence of solutes (which decreases its water potential). Liquid water diffuses from areas of high water potential to areas of low water potential just as heat flows from warm areas to cold areas.
For all organisms the cell membrane and cellular activity allow solute concentration differences to develop between the inside and the outside of the cell, resulting in differences in water purity between the inside of the cell and the outside. When purity differences develop, water will flow in or out by diffusion. As long as the purity differences are small this movement can eliminate the purity differences by making the inside less pure (if water flows out) or more pure (if water flows in). However, such water movement will also cause the cell to change volume and if the cell swells or shrinks too much it can cause irreparable damage to the membrane, thereby destroying cell functioning. As a consequence, organisms without a cell wall must either live in areas where water purity is similar to what is found inside their cells or they must have structures/mechanisms that lessen diffusion and/or have the ability to either eliminate the water that diffuses in (e.g., the contractile vacuole of Paramecium) or to acquire water to replace that being lost by diffusion (generally this is accomplished by acquiring the ‘salty’ water and eliminating the salts).
For cells with a wall (e.g., plants and fungi), the wall allows a new ‘fix’ to living in areas where the cell is more concentrated in solutes than the external environment (this is the normal situation for most non-marine habitats: fresh-water lakes and streams and terrestrial habitats where organisms are immersed partially or totally in soil whose water is generally quite pure, i.e., with few solutes). For these organisms, the rigid wall allows pressure to increase as water flows into the cells. This pressure acts to reduce the inward diffusion of water and eventually a dynamic equilibrium is reached where the high pressure and low purity inside the cell balance the lower pressure and higher purity outside the cell. Water moves (diffuses, i.e., moves by osmosis) in and out at the same rate.
Plants and fungi use ‘osmotic systems’ in a number of ways
Structurally
Water can be used as ‘building blocks’ when it is confined in a structure that will not expand. Living cells, with the combination of a cell membrane and a cell wall, are structurally strong and plants and fungi use them to form rigid structures that can withstand gravitational and wind forces (also discussed in Chapter 3). Evidence for the structural importance of water comes from the observation of wilting: if plants are deprived of a source of water to replace that being lost by evaporation, they loose structural integrity (Fig. 3).
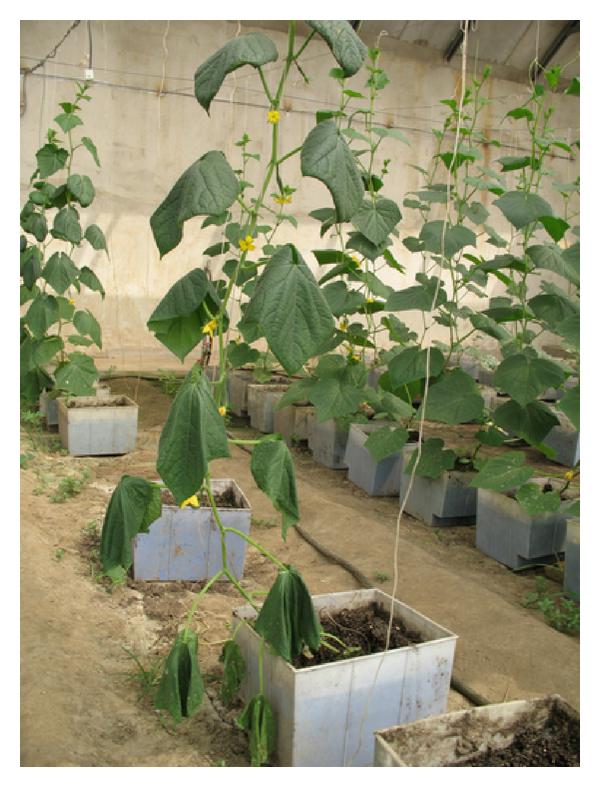
Some plants and fungi produce some structures (e.g., trees, bracket fungi) that don’t collapse when deprived of water, but for many plants access to water is essential to ‘standing up’ because it is the pressurization of cells that provides rigidity. Central to this ability is a cell wall that has high tensile strength and resists expansion and consequently allows for pressurization.
Growth
For organisms with cell walls cellular growth occurs when the internal pressures exceed the strength of the cell wall, causing it to yield to the pressure inside it. Organs (fungal filaments, roots and shoots) grow as a result of the expansion of individual cells and the internal pressure not only has to push out the cell wall but may also have to push away (compress) soil in its path. Thus for plants (both roots and sometimes shoots) and fungi, growth may require the production of a significant amount of force. The force to power this growth comes from the diffusion of water (osmosis), and very significant forces can be created as the result of the movement of water down its water potential gradient. Pressures of 2-4 bars (= 0.2-0.4 MPa [megapascals] = 2-4 atmospheres of pressure = 30-60 pounds per square inch) are common and can be quite effective, as anyone who has observed a dandelion coming up through a sidewalk may have realized.
Guard cells and stomates
The opening and closing of stomates come about as a result of changes in the pressure of specialized cells, guard cells, that surround the pore. Pressurization of the guard cells, as a result of solute accumulation and subsequent water diffusion into the guard cells, causes the cells to swell and form an opening (a stomate) in between them. A decrease in solutes in the guard cells will cause a movement of water out of the cell, resulting in a drop in pressure and consequently stomatal closure. The triggers that stimulate guard cells to accumulate or lose solutes have been extensively studied and include light and the concentration of carbon dioxide. The exact controls may not be the same for all species. At least some plants are able to regulate internal carbon dioxide levels at a ‘set point’ that allows photosynthesis to proceed with little inhibition due to a lack of carbon dioxide, while at the same time minimizing the amount of water lost due to transpiration.
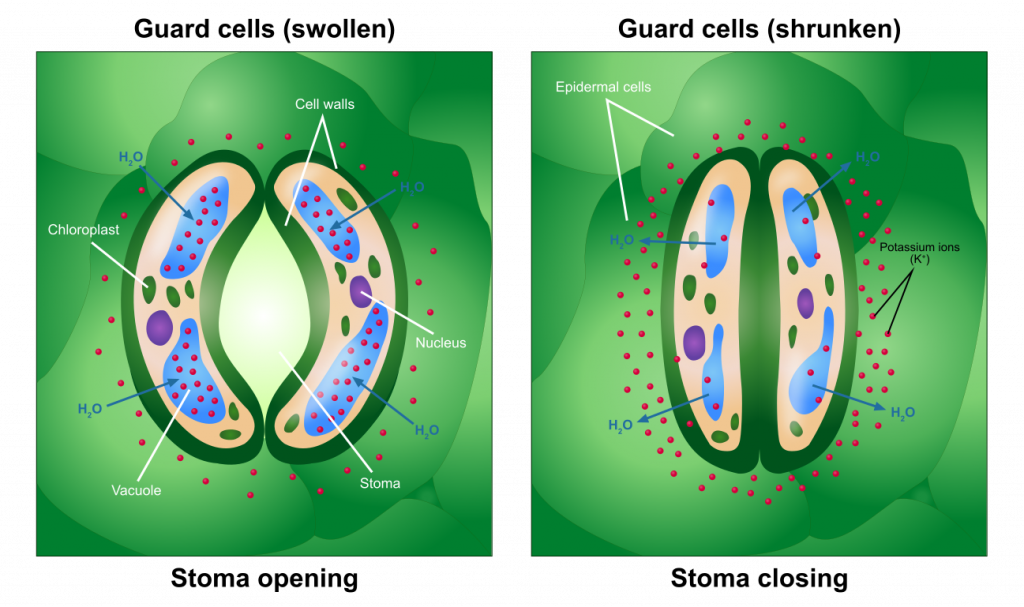
Leaflet movement
Similar to the action of guard cells, a number of plants have leaves or leaflets that move in response to environmental cues such as light, touch and drought, resulting in leaves or leaflets whose orientation varies depending upon circumstances. A common example is ‘sleep movements’ where leaves are horizontal during the day and vertical at night. These movements are the results of changes in the pressure of ‘pulvinar’ cells, located at pivot points. Relatively small changes in the size of these cells are leveraged as a result of their location and can cause substantial changes position of organs involved. (see https://youtu.be/U-PK13JEgk8 below)
Watch
Long-distance transport
Phloem
Both transport systems in plants, the xylem and the phloem, operate as the result of pressure differences created in the ‘pipes’ found in these tissues (see Chapter 6). The pressure differences are created as the result of the diffusion of water. In the phloem, pressures are created as a result of the addition of solutes (sugars) to the pipe cells, a process known as phloem loading, that occurs in regions of the plant called ‘source’ areas. As a consequence of the phloem loading, water flows into the sieve tube elements of the source area, and the pressure increases, triggering a flow in the pipes. The pressure differences and the flow are maintained because solutes are not just loaded at ‘source’ areas, they are also removed at ‘sink’ areas, the locations that solutes are being transported to; thus a continuous pressure gradient occurs in the phloem, from sources that provide sugars, to sinks that consume or store sugars. The exact locations that serve as sources or sinks can change depending upon whether a location is producing sugars (usually sucrose) or consuming them. Phloem transport can be up the plant (e.g., from storage sites in the root to shoot apical meristems) or down the plant (e.g., from photosynthesizing leaves to storage sites in the root). Loading of sucrose requires metabolic energy as ATP is used to move sucrose from where it is less concentrated to where it is more concentrated. As was the case in guard cells, water movement into the sieve tubes is passive once the solutes have been added. Because it is a mass flow, not just the sucrose but any solute that is in the sieve tube will be transported to the sink. The most common of these other solutes are amino acids, but other nitrogen-containing compounds are transported in the phloem along with some mineral elements (e.g., K+, Mg2+, Ca2+)
Xylem
Water transport in the xylem is also the result of ‘pressure’ differences but these are actually differences in tension rather than differences in pressure. While pressure compresses fluids, the tension pulls liquids apart, just as pulling on a string exerts a force that acts to break the molecules of string. Surprisingly, in certain situations, water has substantial tensile strength and can indeed be pulled. The cell walls of all the cells in a leaf are infused and coated with water because of the adhesion of water to the cell walls and the cohesion of water to itself. When water leaves a leaf by evaporation the remaining water is put under tension (sometimes described as ‘stretched’, but the water is not being stretched, rather it is the material that it is attached to), because the remaining smaller volume of water is covering the same original volume of cells. This tension is transmitted to the water in the conducting cells of the xylem and creates a ‘pressure’ difference (actually a tension difference) that can pull water up the (non-living) pipe cells. What is generally driving the water movement up the pipes (tracheids and vessels) is a tension created as water is lost due to transpiration. However, water flow up the xylem can also occur even if there is little transpiration as long as growth is occurring: the diffusion of water into expanding cells can create a tension to pull water up plants in the spring at a time when plants lack leaves and transpiration rates are very low.
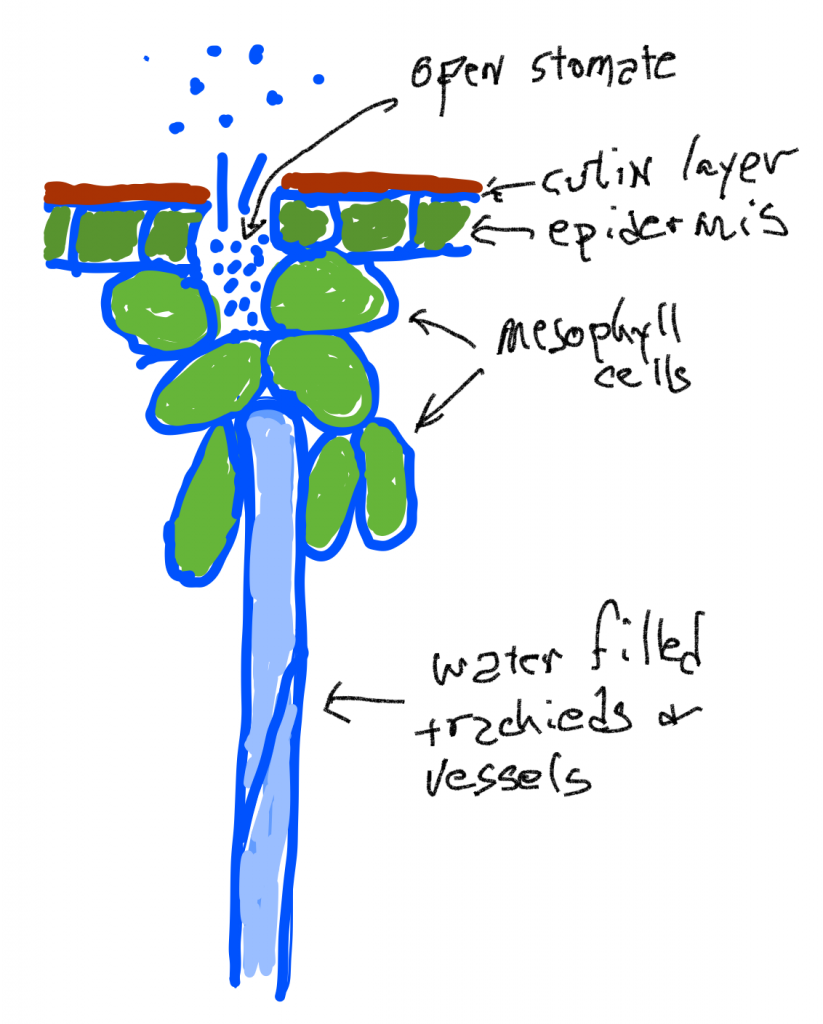
Water loss from the leaf is simple diffusion: water vapor at high concentrations (high humidity of the air inside the leaf) diffuses through open stomata to where the humidity is lower outside the leaf. The humidity of the air inside the leaf is maintained because liquid water in the cell walls of mesophyll cells evaporates and replaces water that has been lost. The tensions generated by water loss causes the tracheids and vessels to be slightly compressed as the pressure outside them is ‘normal’ (one atmosphere) but the pressure inside is lower. Note that this is in contrast to the situation in living mesophyll cells which are pressurized because they have a membrane that allows them to concentrate solutes. If the tension in the water column of becomes too great, a phenomenon called cavitation occurs: air bubbles form when the water column is broken or when water breaks away from the sides of the tracheid or vessel. In either case, the cell is ‘cavitated’ and is no longer useful for water transport.
In both transpiration-driven and growth-driven situations, and in phloem transport, there is a short-distance diffusional movement that creates a pressure difference that can result in a long-distance movement. In both the xylem and phloem the movement within the pipes is NOT diffusion, it is a much more familiar process called bulk flow. Bulk flow is a much more effective means to transport materials over long distances than diffusion, which is only effective over very small distances (tenths of a mm for liquids). Bulk flow is blocked by cell membranes and is impeded, but not prevented, by cell walls. The pits found in the cell walls of tracheids and vessel tube elements provide a relatively low resistance pathway for water to move between adjacent cells. Water flows even more readily through the perforation plates of vessels because the openings are complete (no cell walls). In the (living) conducting cells of the phloem, plasmodesmata connect the individual cells and bulk flow of phloem sap (which is essentially cytoplasm lacking organelles) occurs from cell to cell through the plasmodesmata. Bulk flow is also significant in the soil where there are passageways for water to flow through and where both gravity and the ‘pull’ by movement into plants can create pressure differences.
The pressures and tensions found in vascular tissue reflect these mechanisms. If a sieve tube is penetrated, phloem sap flows out because the pressure inside the cell is greater than atmospheric, just as you will bleed if your skin is severed. Under most circumstances, if a tracheid or vessel is penetrated water does NOT flow out, rather air flows in, reflecting the fact that the water inside the tracheid /vessel was under tension. In fact, if one measures the volume of a tracheid /vessel as tensions develop, it slightly decreases because of compression from the outside. Because of this, tree trunks exhibit a measurable decrease in circumference during the day as transpiration and tensions increase and rebound overnight as the tensions are relieved and the plant is rehydrated during times of little or no transpiration.
On rare occasions the water in the xylem is pressurized.
This condition is described as ‘root pressure’ and only occurs under relatively rare circumstances. ‘Root pressure’ is demonstrated by the ‘bleeding’ (exudation) from a decapitated stem. Under these same special conditions, if one punctures an individual xylem vessel or tracheid it will also bleed, unlike the more normal situation described above. Root pressure occurs if the soil is moist, roots are actively growing, and transpiration is low (at night or when no leaves are present). Under these conditions solutes (mineral ions) accumulate in the xylem of the root because of the actions of root cells, and because the endodermis collectively behaves like a membrane and is a barrier that prevents solutes accumulated in the root xylem from leaking back out of the xylem tissue. Hence, like a living individual plant cell which can pressurize because the cell membrane allows solutes to be accumulated, the entire root xylem can accumulate ions and pressurize. This phenomenon is rare, because solutes typically do not accumulate in the root xylem because mineral acquisition by roots is matched by xylem transport up due to transpiration. Additionally, ‘pull’ from the top (created by transpiration or growth) prevents a pressure buildup from occurring.
A final situation where the xylem is pressurized is the one that causes sap to flow (out) in maple tree trunks in the late winter and early spring. ‘Tapping’, inserting a cylinder, into the xylem, results in the bleeding of sap that can be collected, concentrated and used as a source of sugar. Maple sap flow does NOT require root activity — it can be observed in stems removed from the root system. Maple sap flow DOES require freeze/thaw cycles because these somehow allow the xylem to become pressurized. The sugars found in the xylem sap are coming from xylem rays whose starch is converted into sucrose in late winter. Why only maples and a few other species of trees exhibit this behavior is generally attributed to aspects of their wood anatomy. Most tree species do not pressurize when exposed to freeze/thaw cycles.
Although phloem tissue is penetrated when maple is tapped and although phloem tissue is pressurized and transports sucrose, extremely little of the sap collected from maples is derived from the phloem. The living cells (sieve tube elements) of the phloem tissue are capable of rapidly plugging holes to prevent ‘bleeding’ and consequent sugar loss. If one could tap into the phloem tissue it would be found to have a much, much higher sugar concentration (comparable to maple syrup) than maple sap, whose sweetness is barely detected by humans until it is concentrated. Maple trees that are tapped will bleed from the xylem tissue for several months, as long as they are exposed to proper freeze/thaw and as long as there isn’t a ‘pull’ from the top of the plant caused by growth or evaporation from leaves. Maple sap flows when leaves aren’t present and growth is not occurring.
Further Reading and Viewing
- “Xylem Structure.” Good discussion of xylem transport.
- “Xylem feeding by spittlebug nymphs: some observations by optical and cryo-scanning electron microscopy” by Laura J. Crews et al. What do spittlebugs say about xylem transport.
Media Attributions
- Redwood larger © kern.justin is licensed under a CC BY-NC-ND (Attribution NonCommercial NoDerivatives) license
- Wilting Cucumber Plant © Shenglian Lu, Chunjiang Zhao, Xinyu Guo is licensed under a CC BY (Attribution) license
- Guard cell plant © Ali Zifan is licensed under a CC BY-SA (Attribution ShareAlike) license