Chapter 22: Nutrition and nutrients
In order to grow, an organism has to acquire materials to make itself bigger. These materials are sometimes considered ‘food’ (Fig. 1-2) or may be described as nutrients and this chapter considers what nutrients are required by organisms and how they are acquired. We have already considered three nutrients, carbon, hydrogen and oxygen, that play a role in the energetics of organisms, but they also are important materially. Organisms are built of more than just these three and need the others in order to grow. The acquisition of required materials (i.e., organismal nutrition) is something that distinguishes most of the organisms considered in this book from animals. Nutrition is highly significant, not just to the success (i.e., growth and reproduction) of organisms but also to ecology and to interactions with other organisms and specifically agriculture. For reasons that should be obvious already and that are elaborated on further in this chapter, the nutrition of organisms is strongly affected by their lifestyle, in particular, whether they are an autotroph or heterotroph, and also by their evolutionary history.
TOPICS
- Chemical (molecular) composition of organisms
- Chemical (elemental) composition of organisms
- How nutritional requirements are met by heterotrophs and autotrophs
- Mechanisms of nutrient acquisition
- Vitamins
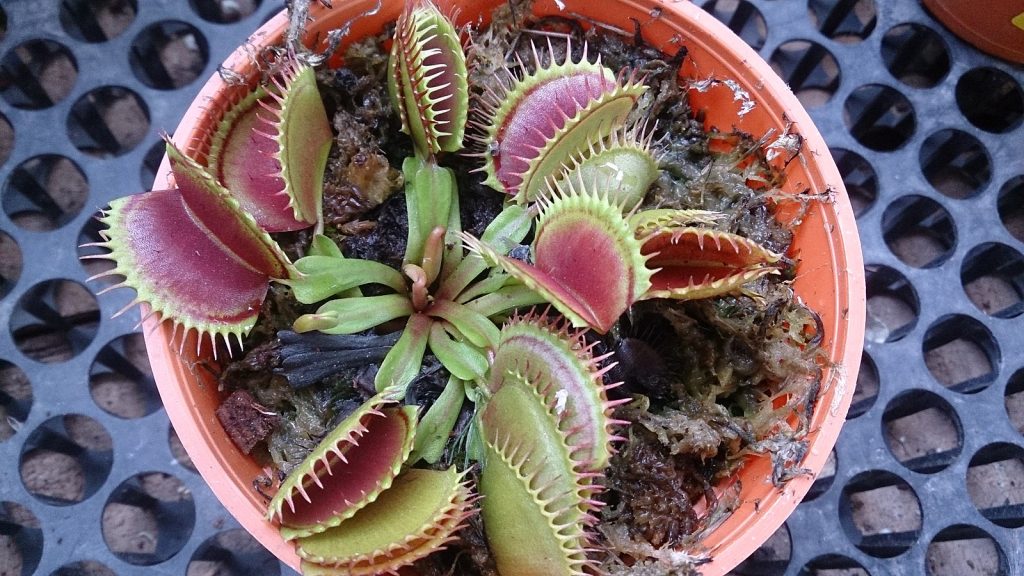
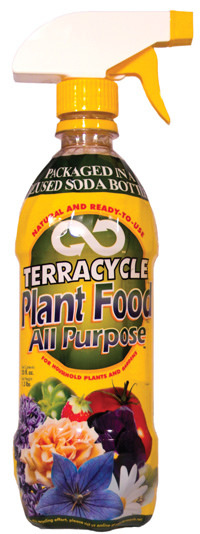
Chemical (Molecular) Composition of Organisms
One of the properties that define organisms is their chemical composition, both in terms of elements (e.g. carbon) and in terms of compounds (combinations of elements, e.g. carbohydrates). The compounds that make up organisms are distinctive in comparison to the world they live in, with most of the chemical compounds (biomolecules) being unique to living things, although some (e.g., silicon dioxide, calcium carbonate) can be produced in non-living situations. A general classification of the molecular composition of organisms is shown below.
type |
chemistry |
elements |
examples |
functions |
carbohydrates | (C6H12O6) | C, H, O | glucose, fructose | metabolites, energy sources |
carbohydrate polymers | polymers of simple sugars | C, H, O | starch, cellulose, hemicellulose, pectin | energy storage, cell wall components |
amino acids | carbohydrate with an NH2 group | C, H, O, N, S | glycine, leucine, aspargine | components of proteins, means of transporting nitrogen atoms |
proteins | polymers of amino acids | C, H, O, N, S | rubisco, DNA polymerase | enzyme catalysts, structural molecules |
lipids | chains of carbon and hydrogen, often with a phosphate attached. | C, H, P | fats, phospholipids, glycolipids, | partitioning the cell into compartments, energy storage in seeds |
nucleiotide | nitrogenous compound attached to sugar plus phosphate groups | C, H, O, N, P | ATP, GTP | energy metabolites, components of nucleic acids |
nucleic acids | polymers of nucleotides | C, H, O, N, P | DNA, RNA | information storage and processing |
Elemental Composition of Organisms
The elemental composition of organisms reflects their molecular composition. Typically around 98% of the mass of any organism is composed of four elements, C, H, O, and N. But other elements are present and many of these are ’essential’, i.e., the organism must have them in order to survive, grow and reproduce. Table 2 lists those elements that are considered essential for all organisms and are also found in high enough concentrations to be considered ‘macronutrients’.
C (carbon) | carbohydrates, lipids, proteins |
H (hydrogen) | everywhere!! also important when present as protons (H+ ) |
O (oxygen) | carbohydrates, proteins, electron acceptor in respiration |
N (nitrogen) | amino acids (proteins), nitrogenous base of nucleiotides, thus in nucleic acids; also present in metabolites such as ATP, NAD, NADP, and many others |
P (phosphorus) | phospholipids, nucleic acids, ATP, and others |
K (potassium) | used exclusively as a cation unbound to an anion; important in effecting changes in membrane charge and influencing water diffusion |
Mg (magnesium) | present in chlorophyll, also an important ion in many enzyme reactions |
Ca (calcium) | A component in cell walls often serves as a messenger in signal transmission, often plays a regulatory role within cells |
S (sulfur) | found in two amino acids, and thus in proteins; also a component of several important metabolites, including some considered as vitamins |
Fe (iron) | found in cytochromes and other iron-sulfur proteins important in the electron transfer processes of both photosynthesis and respiration |
Note that sodium (Na) is not on the list. Sodium is required by animals, where it plays a role in membrane charge, electrolyte balance and nerve transmission. But it is not required by most plants and fungi. In spite of this and because sodium is common in the environment, sodium is generally present in both plants and fungi. Typical sodium concentrations in plants are high enough, in spite of the fact that it is not required, to supply herbivores and other heterotrophs with sodium sufficient for their needs.
In addition to macronutrients, organisms require additional elements but only need them in very small amounts; these are called micronutrients. Table 3 lists micronutrients known to be essential in plants and thought to be essential in all organisms. Table 4 lists micronutrients that are not essential to plants but are thought to be essential to at least some organisms.
Cu (copper) | co-factor in multiple enzymes; in normal (oxygenic) photosynthesis, copper is involved in electron transport between the two photosystems |
Mn (manganese) | a component of several essential enzymes and also in the ‘oxygen-evolving complex’ of the oxygenic photosynthesis process found in plants and cyanobacteria |
Zn (zinc) | required by several enzymes and also is a component of regulatory proteins involved in gene expression |
B (boron) | probably plays a role in several enzyme systems; also present in plant cell walls, important in the elongation of plant cells |
Cl (chlorine) | important as an electrolyte; in plants, chlorine is also involved with water splitting and the oxygen-evolving complex of oxygenic photosynthesis |
Ni (nickel) | involved several essential enzymes |
Mo (molybdenum) | involved in several essential enzymes; in plants, Mo is essential to processes involved with nitrogen assimilation |
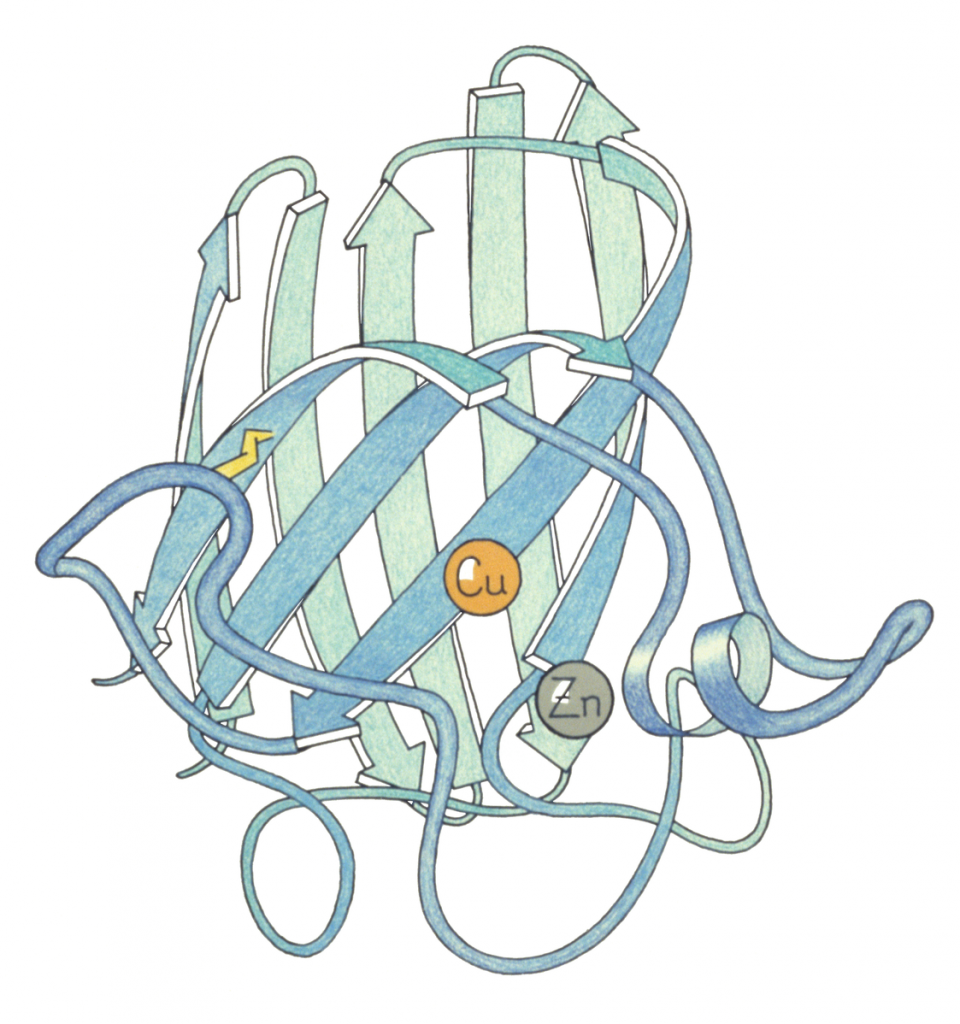
Na (sodium) | not required for most plants but commonly present in them; required by animals where it plays a role in electrolyte balance, membrane charge and nerve transmission |
I (iodine) | a component of thyroid hormones, not required by plants |
Co (cobalt) | a component of vitamin B12, which is essential for animals and many protists (including some photosynthetic protists), also required by bacteria and cyanobacteria that carry out nitrogen fixation |
Se (selenium) | part of several enzymes, including some that eliminate oxidant molecules, required by few plants where it is generally is a component of antiherbivore compounds |
Vn (vanadium) | component of antioxidant enzyme found in diatoms and red, brown and green algae; needed in nitrogen-fixing bacteria and cyanobacteria |
Cr (chromium) | essential role debated, some cite a role related to insulin |
Fl (fluorine) | essential role debated but is known to strengthen bones and teeth |
As (arsenic) | essential in rats and mice; essentiality not established for humans, role not known |
Sn (tin) | essential in rats and mice; essentiality not established for humans, role not known |
Tables 2 and 3 list the 17 essential elements required by plants. The elemental composition of plants, and organisms in general, is not reflective of the abundance of elements in the atmosphere (80% nitrogen, 18% oxygen) or the solid earth (46% oxygen, 28% silicon, 8% aluminum, 5% iron ~ 3% calcium, sodium, potassium, magnesium) or dissolved in the water (chlorine 19.1 g/kg of seawater, sodium 10.7 g/kg, magnesium 1.3 g/kg, sulfate 2.7 g/kg). Clearly, organisms are not in equilibrium with their environment and somehow acquire elements to higher levels than found in their environment.
Acquisition of nutrients—how nutritional needs are met
Nutrition is strongly influenced by lifestyle. For heterotrophs, their chemical composition and how they acquire it are relatively easy to explain — ‘you are what you eat;’ the composition of heterotrophs reflects their absorption of biological molecules that are derived from the organisms that they ingest. And since all life is made of the same materials, the consumption of biomolecules by heterotrophs should allow heterotrophs to acquire the material required to make more of themselves and consequently to grow. Recall that some heterotrophs are ‘ingesters’ (e.g., lions, caterpillars, humans) swallowing organisms or parts of organisms into a tube inside their body where digestion occurs that breaks down the large biological molecules (e.g., proteins) into smaller ones (amino acids) that can be absorbed by the organism. Other heterotrophs are ’absorbers’ (e.g., fungi, many bacteria, water molds) and do the digesting outside of their body. The basic process is the same in both groups: large molecules are broken down into smaller ones that can be absorbed. Three key factors influence the nutrition of heterotrophs: food choice (what they choose to eat), digestive abilities (what biomolecules they can break down into smaller units, and absorptive abilities, what molecules they can transport into their cells. These factors vary tremendously, especially within the archaea, bacteria and fungal groups. Also note that for absorbers, digestion may be partly or largely the result of other organisms living in the same habitat. A similar situation exists within ingesters because they usually harbor organisms within their gut track that participate in digestion. Note that for heterotrophs the acquisition of the nutrients shown in Table 4 may be problematic because the element may not necessarily be in the ingested food, e.g., iodine is not required by plants so heterotrophs may not necessarily acquire it from the food they eat. The same is potentially true for sodium, but in practice, most plants contain sodium even though they do not require it because sodium is common in the environment.
The nutrition of autotrophs is very different. They need the same elements that heterotrophs do (Tables 2 and 3) but they do not acquire these in a ‘prepackaged’ form. Moreover, many autotrophs can’t utilize nutrients in a prepackaged form. While some photosynthetic protists (algae) and many photosynthetic prokaryotes do have the ability to absorb organic compounds, plants have no ability to ingest materials (i.e., no mouth and digestive tract), nor can they break down large organic molecules outside their body, or even to absorb breakdown products like amino acids, should they happen to be present. In fact, the materials that plants absorb are not ‘organic’ (= biological), they are elements or simple compounds found in the environment, such as CO2 (carbon dioxide), NO3– (nitrate), SO42- (sulfate) , PO4– (phosphate) or elemental ions (e.g., Ca2+, Cl–). Autotrophs have the ability, which heterotrophs lack, of transforming these elements and simple compounds into biological compounds. By far the most extensive process is the conversion of carbon dioxide and water into carbohydrates, but many other reactions are essential: adding nitrogen groups to carbohydrate molecules to produ ce amino acids, synthesizing nucleotides, nucleic acids, assorted metabolites (e.g., NADP+, assorted vitamins), etc. This process is made all the more challenging by the fact that the raw materials used by autotrophs are all dilute and scattered in the environment. This is in contrast to the materials that heterotrophs consume, where all the needed materials are usually found together in ‘food’.
In order for autotrophs to acquire the minerals, they need these minerals must be in a form that dissolves in water and in a form that autotrophs can acquire (i.e., will pass through a membrane or through a channel/carrier protein imbedded in a membrane). For aquatic autotrophs, all of the nutrients that they acquire come from the solution they are immersed in. For terrestrial autotrophs (plants), carbon is the sole element acquired directly from the air, as carbon dioxide. All other nutrients come from the ‘soil solution’, the water held in the soil. Not only must nutrients be in soil solution, they also have to be in a form that the organism can assimilate. For example dinitrogen gas (N2), readily dissolves in water, and readily enters into organisms, but it can only be assimilated, i.e., incorporated into an organic form, by a relatively small group of organisms in both the Bacteria and Archaea groups. Plants, other eukaryotic autotrophs, and most prokaryotic autotrophs cannot assimilate N2 and need to acquire nitrogen as either ammonia or nitrate.
The role of heterotrophs in autotroph nutrition
A common bumper sticker used to be ‘Have you thanked a green plant today?’— the message being that green plants are essential to all life on earth because they form the base of the food chain. A comparable message is also significant—’ Have you thanked a heterotroph today?’ As essential as green plants are, they in turn are dependent on heterotrophs. Heterotrophs are essential to autotrophs (and thus to heterotrophs themselves, i.e., this is circular) because heterotrophs put nutrients into a form that autotrophs can use. Without heterotrophs, green plants would be unable to acquire the carbon and other elements that they require. When viewed by autotrophs the general role of heterotrophs is decomposition, to break down organic material into simple ‘inorganic’ compounds that they can utilize, a process described as ‘mineralization’.
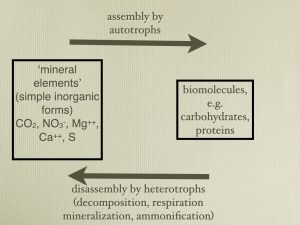
Because nutrients are diluted in the environment and concentrated in organisms, absorption of elements involves accumulation — the concentration of elements inside organisms. And this accumulation requires energy and is an ‘active’ process. Some nutrients are absorbed in the charged (ionic) state. Since the inside of the cell is negatively charged relative to the outside of the cell, nutrients that are cations (positively charged) may actually be accumulated by moving down their electrochemical gradient. In such a situation the accumulation might be considered ‘passive’ (down an electrochemical gradient) but this is ignoring the fact that energy is required to make the inside of the cell negatively charged.
For both autotrophs and heterotrophs, a variety of cellular mechanisms account for the absorption of nutrients. They include the following:
- diffusion across the membrane: since most nutrients are charged and since charged molecules do not readily penetrate the phospholipid bilayer of the cellular membrane, this is relatively rare; however, occasionally it can account for the absorption of uncharged molecules, e.g., ammonia (NH3)
- passive diffusion through channels: Cations are sometimes acquired by diffusion down an electrochemical gradient through protein-structured ‘pores’ in the phospholipid bilayer. Generally, these pores are selective and only allow certain cations to pass through them.
- pumps: some nutrients move across the membrane in a process that requires an energy source, typically ATP. This movement involves proteins (enzymes) whose conformation changes in response to some process that is energetically driven. Generally, pumps account for the accumulation of nutrients against an electrochemical gradient, but they could also could also account for enhanced movement of cations down their electrochemical gradient.
- coupled ion transport: Since cells are negatively charged, the movement of charged molecules can be coupled to the passive movement of cations into the cell, or anions out of the cell. Most commonly, anion movement into the cell is coupled with the inward movement of protons. Coupled ion transport also involves proteins and generally is quite specific with regard to nutrients, i.e., there are separate carriers for different ions.
Absorption mechanics are significant because all of the elements required by autotrophs may actually become toxic (with levels high enough to inhibit growth) if they become overly abundant in the environment (e.g., chlorine toxicity in saline soils). It is also significant that autotrophs often take up elements that they do not require and this may be beneficial (e.g., sodium) or detrimental (e.g., arsenic) to the heterotrophs down the food chain.
Acquisition mechanisms for essential nutrients
Carbon
For all autotrophic organisms carbon is acquired as carbon dioxide, either from the atmosphere or dissolved in water. Carbon dioxide dissolves in water and then can be transformed into a variety of compounds by (mostly) abiotic chemical reactions. The most significant reactions are the formation of carbonic acid from carbon dioxide and water; the formation of bicarbonate ion as carbonic acid loses a proton; the formation of carbonate ion as bicarbonate ion loses a proton. Carbon is readily exchanged between these pools and all of them can be considered ‘biologically active’ forms of carbon.
H2 O+ CO2 — > H2 CO3 (carbonic acid)
H2 CO3 — > H+ + HCO3– (bicarbonate ion)
HCO3– —> H+ + CO32- (carbonate ion)
For heterotrophic organisms, carbon is acquired in a variety of biomolecules: carbohydrates, proteins, lipids. The exact mix of compounds depends on the dietary preferences of the organism. Heterotrophs can only absorb relatively small molecules (simple sugars, amino acids, nucleotides) and therefore often have to break down polymers (e.g., starch, proteins) before absorption actually occurs. Once inside the cells of the organism, these small molecules (e.g., glucose, amino acids) may be oxidized in cellular respiration, decomposing them to carbon dioxide, water, and (for amino acids) some nitrogenous compounds like ammonia. A bsorbed nutrients may also be ‘reassembled’ into polymers or metabolites like NADH that are used for growth or to replace molecules that have been broken down.
Hydrogen
For autotrophs, hydrogen is is acquired in water molecules and occasionally in other compounds. For heterotrophs, hydrogen is acquired in water, carbohydrates, proteins and lipids.
Oxygen
Oxygen plays two roles in organisms: a structural role, being a part of most biomolecules (carbohydrates, proteins, nucleic acids) and a dynamic role, being an essential reactant in cellular respiration that is subsequently lost as water. For autotrophs, oxygen for the structural role is acquired as carbon dioxide that is incorporated into carbohydrates and subsequently into other important biological molecules. For heterotrophs, structural oxygen is acquired in the food that they consume. For both autotrophs and heterotrophs, oxygen for respiration is acquired as molecular oxygen (O2) which, for terrestrial organisms, can be acquired directly from the atmosphere where it accounts for nearly 20% of the air’s molecules. In aquatic systems, oxygen is obtained from the water where it usually is present as a dissolved solute. Oxygen concentrations in water are variable, depending primarily on exchange with the atmosphere, biological activity and how much the water is being mixed (circulated). Photosynthetic organisms produce oxygen but this effect is limited to the region of the water column that receives light. Throughout the water column, oxygen is consumed by all aerobic organisms. How much the oxygen levels are lowered by this action depends on the amount of living things, their rate of oxygen consumption (this is a strong function of temperature), and the rate of oxygen delivery to the system.
Nitrogen
For heterotrophs, nitrogen is obtained from the food that they eat, primarily from proteins, but also from nucleic acids and nucleotides. Some fungi, bacteria and archaea can acquire nitrogen as nitrate (NO3–) or ammonia (NH3) or ammonium ion (NH4+). For plants, nitrogen is always acquired as nitrate or ammonia dissolved in water. Although nitrate and ammonia are considered to be ‘inorganic’ molecules they are almost always produced from biological molecules as a consequence of the following biological processes:
ammonification is the production of ammonia and can be considered to be a type of ‘decomposition’. It occurs as amino acids are used as a source of energy; the carbohydrate component of amino acids is oxidized in cellular respiration and the amino group is either directly excreted as ammonia or as some other small nitrogen-containing molecule (urea, uric acid). All heterotrophs participate in ammonification, either by directly producing ammonia, or indirectly by producing compounds like urea and uric acid that are readily converted (mostly by bacteria) to ammonia. How much a particular heterotroph participates in ammonification depends upon their diet, specifically how much protein they consume.
nitrification is the production of nitrate. Nitrate is produced by the action of a small group of chemosynthetic organisms (previous chapter) that use ammonia as a source of energy; as ammonia is oxidized to nitrate, an electron flow is created that can result in the synthesis of ATP. Additionally, some chemosynthetic organisms use the reducing power of ammonia to reduce carbon dioxide to carbohydrate. The process first involves the conversion of ammonia to nitrite (NO2–) by one group of bacteria followed by the conversion of nitrite to nitrate (NO3–) by a second group of bacteria.
Ammonia is uncommon in most soils because it is usually quickly converted to nitrate by nitrifying bacteria and also because it is volatile and can be lost from the soil by vaporization, unless acidic soil conditions transform it to ammonium ion, which is not volatile.
Most plants take up nitrate more readily than ammonia, but a few prefer ammonia. Ammonia is a toxic compound and is rapidly metabolized by plants (and other organisms) if it is absorbed.
The pools and processes of the nitrogen cycle are illustrated in the figure (Fig. 5) and table (Table 5).
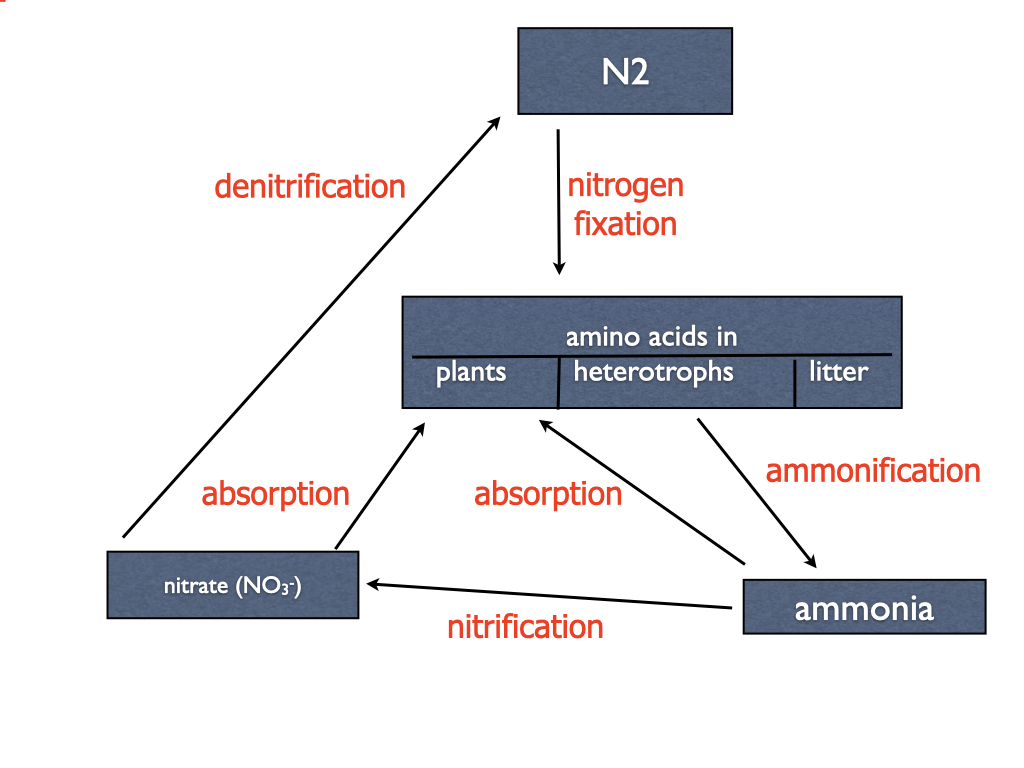
Process | Who does it? | Chemical notes | |
---|---|---|---|
1 | nitrogen fixation | only a few prokaryotes, including Nostoc and Rhizobium | nitrogen is reduced, electron source ultimately is carbohydrate |
2 | consumption | all heterotrophs | no change in chemical form; proteins broken down to amino acids and then used to make more proteins |
3 | death | all life | no change in chemical form |
4 | ammonification (respiration/excretion) | most heterotrophs, especially if they consume protein rich food | oxidation of amino acids forms poisonous ammonia which must be excreted |
5 | nitrification | a very small group of prokaryotes, called nitrifying bacteria | ammonia serves as an electron donor; electron flow to oxygen allows ATP to be synthesized (chemosynthesis) |
6 | absorption | most plants absorb nitrate, a few prefer ammonia | if nitrate is absorbed the plant must reduce it to NH2 to to form amino acids |
7 | denitrification | a very small group of obligate anaerobic prokaryotes, called denitrifying bacteria | nitrate substitutes for oxygen as an electron acceptor for anaerobic conditions |
8 | symbiotic nitrogen fixation | bacteria + plants | bacteria symbiotically associated with plants absorb N2, form ammonia and transfer it to host plants |
Phosphorus
For heterotrophs, phosphorus is obtained as phospholipids, nucleic acids and other metabolites in the food that they acquire and break down. For autotrophs, phosphorus is generally acquired as the phosphate anion (PO4–) which is made available by the action of heterotrophs who break down organic material and release phosphate. Phosphate is a key nutrient in aquatic systems and often regulates the amount of autotroph biomass and primary production.
Calcium, magnesium and potassium
These ions play multiple roles in organisms, generally being present as dissolved cations but occasionally being permanent parts of molecules (e.g., magnesium is part of the chlorophyll molecule). For heterotrophs, these elements are obtained as cations dissolved in the cytosol of the cells that they digest. Plants absorb Ca2+, Mg2+ and K+ as cations dissolved in the soil solution. These ions are generally derived from the breakdown of organic material (decompositions) and as soil minerals are weathered (dissolved) and put in solution. Fungi are unusual compared to most eukaryotes because they generally require much lower levels of calcium.
Sulfur
For heterotrophs, sulfur is obtained primarily from protein digestion releasing the two sulfur amino acids, cysteine and methionine and their subsequent absorption. While some heterotrophs can utilize either cysteine or methionine as a sulfur source, humans and some other animals cannot synthesize methionine from cysteine and therefore methionine must be obtained in their food. For autotrophs, sulfur is acquired as the sulfate anion (SO4–) and subsequently needs to be reduced to produce all biologically active forms.
Iron
For heterotrophs, iron is obtained from organic material, where it is a universal cellular constituent, albeit in low concentrations. Iron absorption into the heterotroph is sometimes deficient and iron deficiencies may result from an inability to absorb rather than from a lack of iron in food. For plants, iron is acquired as both the ferrous (Fe+2) or ferric (Fe+3) ion. The ferrous ion is much more soluble in water but is much less common under normal (high oxygen) conditions which cause iron to be in the more oxidized ferric state. In this state, the availability of iron is strongly influenced by pH, with less available iron at higher pH’s (over 6). Consequently, although iron is very common in soils it is often unavailable to plants because it is not in solution, especially at high pH’s. However if the soil becomes waterlogged and anaerobic, iron becomes readily available in the ferrous state and may become toxic.
Sodium
Sodium is common in most water sources, even ‘fresh water’ sources. While sodium is known to be essential for few plant species, in particular many CAM and C4 plants, it is not essential for most plants. In spite of this, sodium does promote the growth of a number of plants. At the sometime, like most plant nutrients, sodium is can be toxic to plants when at high concentrations. Although typical soils do not have sodium concentrations high enough to cause problems, arid regions commonly do have ‘saline soils’, soils with high concentrations of sodium ion in the soil solution. Additionally, irrigation can cause an accumulation of sodium, and adversely affect plant growth because irrigation delivers solutes, including sodium to farmland. Not surprisingly plants do not have active mechanisms to acquire sodium, nonetheless, especially in saline soils, it does ‘leak’ in. Plants do have mechanisms to excrete sodium and also to sequester it in vacuoles. As mentioned previously sodium is generally present in plants amounts sufficient to supply the needs of most heterotrophs.
Micronutrients (molybdenum, chlorine, boron, copper, zinc, manganese, nickel)
For both heterotrophs and autotrophs these elements are absorbed either in elemental ionic forms (Zn2+, Mn2+, Cu2+, Ni2+, Cl–) or as simple molecules (MnO42-), H3 BO. Because most of these ions/compounds are not only essential but can become toxic, control of their movement is important and most, perhaps all, have specific protein transporters that control uptake, movement and sequestration.
Vitamins
A final nutritional category is ‘vitamins’. These are molecules, not elements, that play a critical role in specific chemical reactions and, for a variety of reasons (inability to synthesize, inability to absorb, metabolic disorders), may be deficient in certain situations. Bacteria and archaea, whether autotroph or heterotroph, do not have vitamin requirements, generally because they make these metabolites themselves from the ‘raw materials’ that they require, or, less commonly, they have alternative biochemical pathways that avoid the vitamin requiring step. (There are, however, multiple bacterial strains found and/or developed that have specific vitamin or other nutritional requirements. These have been very useful tools for research). Among eukaryotes, plants, like prokaryotes, generally have no vitamin requirements, generally because they can synthesize vitamins along with the many other molecules that they manufacture. In contrast, eukaryotic heterotrophs often do have vitamin requirements, i.e., molecules that they cannot make and therefore that they must acquire from the food that they eat. For example, humans, and a few other animals, require vitamin C because they are unable to synthesize it. Plants and most animals, including cows, make vitamin C. But although cows can make the vitamin, meat provides very little to consumers, so a diet containing fruits and vegetables is important in preventing vitamin C deficiency in humans. Among other roles, vitamin B3 (niacin) is a precursor to the metabolites NAD and NADP discussed earlier. Although humans and other animals can make niacin from the amino acid tryptophan, they can develop niacin deficiencies if eating a diet low in niacin and tryptophan. Plants can make both niacin and tryptophan from mineral elements and the carbohydrates produced in photosynthesis. They therefore never have niacin deficiency and can provide these nutrients to heterotrophs that consume them. The vitamin requirements of heterotrophs apparently reflect a loss of metabolic abilities through evolutionary time, presumably the result of the fact that the vitamins are generally present in the organic matter that they consume. Plants, who do no consuming, must manufacture any required metabolite. Surprisingly, a number of autotrophic algae groups do have vitamin requirements for several B vitamins. It probably is significant that these organisms are aquatic and B vitamins are water-soluble and hence commonly found in aquatic environments, the result of decomposition of organic matter. In some cases the requirement for B vitamins in some algae may reflect their heterotrophic ancestry.
Further Reading and Viewing
- “Genlisea: A carnivorous plant acting as a trap for Protozoa” by Wilhelm Barthlott et al. Genlisea, an unusual carnivorous plant.
- “Insights into the Evolution of Vitamin B12 Auxotrophy from Sequenced Algal Genomes” by Katherine E. Helliwell et al. Vitamin B12 requirements.
- “Algae need their vitamins” by Martin T Croft et al. Algae need their vitamins.
Media Attributions
- Venus Fly trap © Mokkie is licensed under a CC BY-SA (Attribution ShareAlike) license
- Terracycle © Terra cycle is licensed under a CC BY-SA (Attribution ShareAlike) license
- Ribbon Model © Dcrjsr is licensed under a CC BY (Attribution) license
- Nutrient cycles
- Nutrient cycles