Chapter 5: Cellular Structure in Inanimate Life
The discovery of the first cell is often attributed to Robert Hooke in the middle of the 17th century. But people had observed cells before (fish eggs, frogs’ eggs, birds’ eggs). What was significant about Hooke’s observation was that he noted that organisms, at least some of them, were composed of smaller entities that he called cells. He was able to see them in part because he was using the recently developed microscope. But what also made the cells visible were that they were plant cells, cells that not only are larger than most animals cells but also have cell walls that outline each cell, are rigid and do not collapse when the material is cut into thin sections. The material he was looking at was cork, the outer bark of a tree, and what he was obser ving was the remnants of cells that were no longer alive; their existence was preserved because of their thick and persistent cell walls (Fig. 1).
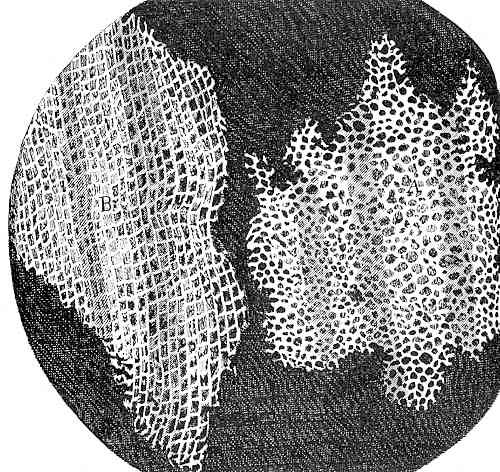
While Hooke’s observations illustrated that at least some parts of some organisms were composed of smaller units, the generalizat ion that all organisms were composed of one to many ‘fundamental units’, cells, was not to be fully realized for another 200 years when ‘cell theory’ was proposed and championed. Although people were very aware of fish eggs and frog eggs, they had not appreciated that when the eggs turned into tadpoles the material was first divided up into many, many small units, each with the same basic construction. With time, these units diversified from each other and transformed into groups to serve specific roles. These are arranged in a particular way to produce a complex whole, a frog embryo. Think of making castles out of legos. A similar transformation occurs as the fertilized egg of plants transforms into a plant embryo, although the egg cell of plants (about 20 um) is 100 times smaller than those of frogs and about five times smaller than those of humans.
One of the great themes of biology is the unity and diversity of life: how organisms can simultaneously be so similar and so different. The same concept also applies to cells and is fundamental to ‘cell theory’: living things are made up of cells, whose basic organization, chemical composition and organization is similar; but these cells are also diverse in terms of shape and function and the diversity is seen both between different organisms (plant cells are different from animal cells) but also within (multicellular) organisms.
Diversity of cells within an organism is one, and for some people the only, criterion for a multicellular organism, not simply that they are made of multiple cells but that at least some of the cells differ from one another. Before describing the diversity of cell types found in some of the organisms that we cover, we will consider additional criteria that might be used to define multicellularity.
TOPICS
- Multicellularity
- Cell Structure of Fungi
- Chytrids
- Coenocytic, filamentous fungi — bread molds and Glomeromycota
- Septate fungi — Club and sac fungi
- Cell Structure in water molds
- Cell structure in algae
- Brown algae
- Red Algae
- Green algae
Multicellularity
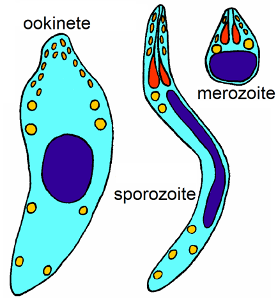
Can a unicellular organism be multicellular? One might think that this is absurd, yet it can be true, depending on definitions. An illustrative case is the Apicomplexa, a group that includes is the causal organism for malaria, Plasmodium. Members of this group are always unicellular but have multiple cell types (Fig. 2), cells that look different, behave differently and live in different places. Most biologists would not consider the Apicomplexa to be multicellular and would rather consider it as a biological entity with multiple forms, but in a developmental sense it raises the same question that multicellularity poses: if one cell is identical genetically to another cell, how can they end up looking and behaving differently?
If you study the life cycle of Plasmodium carefully you will note that some of the cellular diversity is associated with reproduction and the sexual process, a topic that will be dealt with in more detail later but for now simply note that most organisms produce different cells that are associated with reproduction and/or sex. Chlamydomonas, a unicellular green algae (Fig. 3) , produces bi-flagellate cells that all look and behave alike except for: (1) some cells look different because they lose their flagellae and divide twice to produce four cells while still within the parental cell. Eventually the parental cell wall is digested and the four typical-looking cells are released, (2) some cells look normal but are ‘special’, functionally different, because they are able to fuse with other ‘special cells’. Cells become ‘special’ because of changing conditions, e.g., nutrients become less available. (3) the cell formed by the fusion of two special cells develops into a very different looking cell, one with no flagellae and a thick cell wall. It also functions differently, because it is metabolically inactive. Eventually it becomes active and divides to produce four typical looking (bi-flagellate) daughter cells that are released after the cell wall is digested and weakened. These three atypical cell types are associated with sex and the process of meiosis (Fig 3, right-hand side of the diagram) will discussed in more detail later, along with a consideration of reproduction (left-hand side of Fig 3).
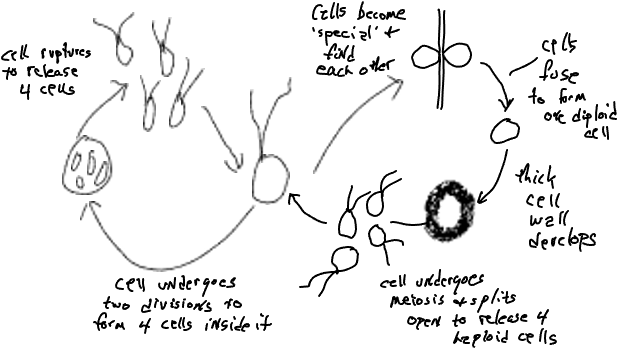
Obviously very few would consider unicellular organisms like Plasmodium or Chlamydomonas to be multicellular in spite of the fact that they may exhibit multiple cell types. The point is that the possession of different-looking or different-behaving cells (e.g., egg, sperm, zygote, spore) that are associated with the reproductive or sexual process are not sufficient to label an organism as multicellular. This is trivially true for unicellular organisms but is also true for colonial organisms that are made up of multiple cells that are almost all the same and is the reason for the distinction between ‘simple multicellularity’ and ‘complex multicellularity’.
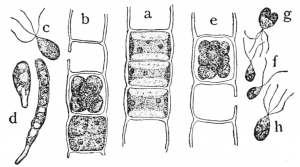
Consider the filamentous green algae Ulothrix, (Fig. 4) which exists as strings of cells, most of which all look alike (see the center part of the drawing labelled ‘a’), but sometimes some of the cells transform (i.e., develop) to produce mobile cells that have four flagellae (‘c’ in the diagram) and are released from the filament. These cells can establish new filaments by attaching to a substrate and dividing to produce new filament (‘d’). Other cells transform and produce and later release cells with only two flagella (‘f’). These cells must find another bi-flagellated cell, fuse with it (‘g’), then undergo meiosis to form four quadriflagellate cells (‘h’) that can attach to a substrate and form new filaments.
Another example of simple multicellularity with diverse cell types associated with reproduction and sex is found in Oedogonium, (Fig. 5) another filamentous green alga. Most cells are cylindric with lengths about five times longer than their width. But there are also egg cells that are shorter and bulge in the middle, and there are much shorter sperm producing cells, with lengths the same or shorter than their width. Both these cell types develop holes in their walls that allows the short cells to release mobile sperm and allows the sperm to enter into the egg cells, resulting in the production of cell with genetic information from two parents. This develops into a dormant spore (zygospore) that is eventually released from the filament. An additional cell type can develop from the normal cells as they develop flagellae and eventually are released from the filament as cells capable of forming new individuals.
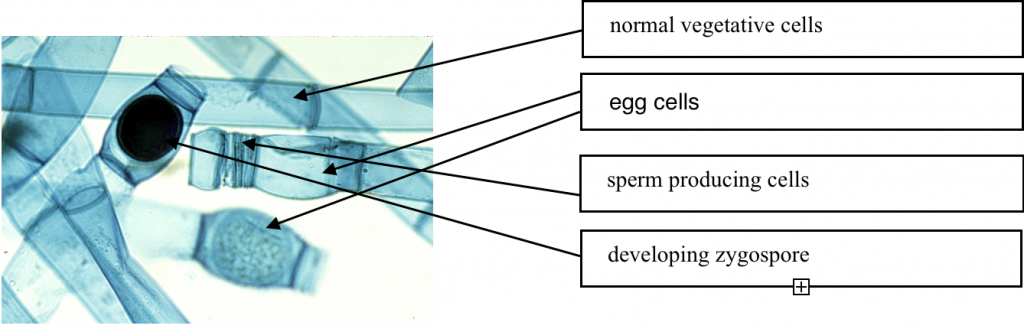
These examples demonstrate common cell types associated sex and reproduction and found in organisms with colonial growth (showing ‘simple multicellularity’): (1) cells that are capable of fusing with each other, called gametes that are sometimes differentiated into egg and sperm, (2) zygote, a cell formed by the fusion of gametes, (3) zoospores, flagellated cells specialized for mobility (dispersal in space) (e.g. the cell labelled ‘c’ in the Ulothrix diagram), (4) inactive, dormant cells that allow for dispersal in time. In general, these are called spores (but note that zoospores are NOT inactive). These inactive cells are called endospores in bacteria and akinetes in cyanobacteria. In a number of organisms (e.g., Chlamydomonas, Oedogonium, bread mold) spores develop from zygotes and are called zygospores. We will see a number of different types of spores in the fungi.
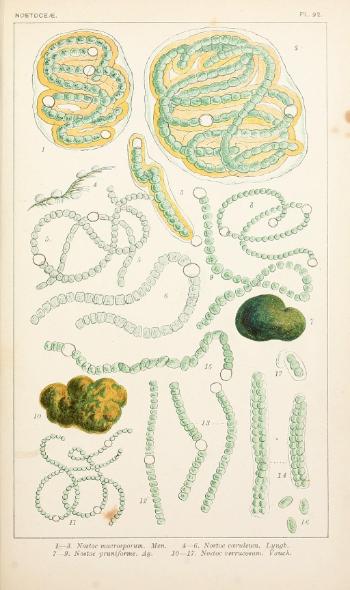
To repeat, none of the examples considered so far would be considered multicellular even though they produce multiple cells, some of which are very different structurally and functionally from others. What additional features contribute to the case for ‘true multicellularity’? One feature is mutual dependency. Most of the cells of Anabena, a cyanobacteria, have chlorophyll and photosynthesize to feed themselves. Anabena also produces cells called heterocysts that lack chlorophyll and are unable to photosynthesize. They are ‘fed’ by adjacent, ‘regular’ cells. Heterocysts are significant because they can take dinitrogen gas, a form of nitrogen that ‘regular’ cells cannot utilize, and convert it into a form that the regular cells can utilize (more details in Chapter 22). Hence, the heterocysts and the regular cells depend on each other. At least some workers would consider the dependency of one cell type on another to be a criterion that makes Anabena multicellular. Another feature that Anabena demonstrates and that is significant to the concept of multicellularity is material movement (food, nitrogen compounds) between the cells of the organism. This really is a form of intercellular communication, and many workers consider intercellular communication to be a key requirement defining multicellularity. Features that enhance communication include trans-cellular connections, plasmodesmata in the case of plants.
Another criterion that has been applied to define ‘true multicellularity’ is a three-dimensional cellular organization, i.e., not just cells added continuously (without and endpoint, i.e. indeterminate development) in one dimension (a filament) or added in two dimensions (a sheet), or added in three dimensions. True multicellularity to some workers requires a developmental program that produces a distinct three-dimensional form that requires that the size, shape and activities of cells be influenced by a combination of location (where they are in the organism) and where they came from (cellular heritage). Such a structure requires extensive communication and coordination between cells. Note, however, it does not necessarily require tissues made up of different cell types.
Workers who study the evolution of life believe that simple multicellularity (colonies) has appeared at least twenty-five times. Complex multicellularity (or what some would call ‘true’ multicellularity), but defined without the requirement of multiple cell types and tissues, has evolved at least ten times: once in the group that led to animals, once in the green algal group that led to plants, once in another green algal group that did not lead to plants, twice in the red algae, twice in the brown algae, three times in the fungi, and several times in the prokaryotes. If the definition of multicellularity requires tissues with multiple cell types (not associated with sex and reproduction) then the number of multicellular groups is much reduced: just animals, plants and a very few fungi.
The cellular structure of select groups of inanimate organisms, organisms that might be considered multicellular, is described below. Excluded are the vascular plants; these are considered in the next chapter.
Cellular Structure in Fungi
While many biologists characterize the fungal group as being ‘multicellular’, many clearly are not. Three fungal groups are coenocytic and none of these are easily characterized as being multicellular. And unicellular fungi, which exist in several fungal groups, would certainly not be considered multicellular. The fungi that might be considered as possessing complex multicellularity do so for only a small portion of their existence and only in a small portion of their structure. Almost all fungi produce a filamentous structure, a hypha (plural hyphae), that is not multicellular and most fungi are better described as being colonial, a filamentous organism with no specialization of cells whatsoever. In three of the fungal groups the hyphae lack cross walls and are coenocytic but the two largest groups of fungi do produce cross-walls (Fig 7) and exhibit simple multicellularity. Hyphae grow from the tip and can bifurcate to branch in two. Branching can also occasionally occur away from the tip. Hyphae may also fuse with one another, thereby producing an anastomosing structure. The network of hyphae thus formed is termed a mycelium. The typical mycelium is a feeding structure with a dynamic (growing and dying simultaneously), diffuse, form that is well suited to obtain nutrients: it possesses both a great deal of surface area for absorption and it also penetrates a large environmental volume, allowing it to ‘mine’ a substrate for nutrients. The feeding mycelium is the digestive system of fungi and simultaneously serves as: (1) a structure to digest food, (2) a ‘small intestine’ to absorb nutrients (3) a circulatory system to move nutrients to other parts of the fungus and ultimately to reproductive structures. Note that none of these functions are associated with specific cell types, tissues or organs.
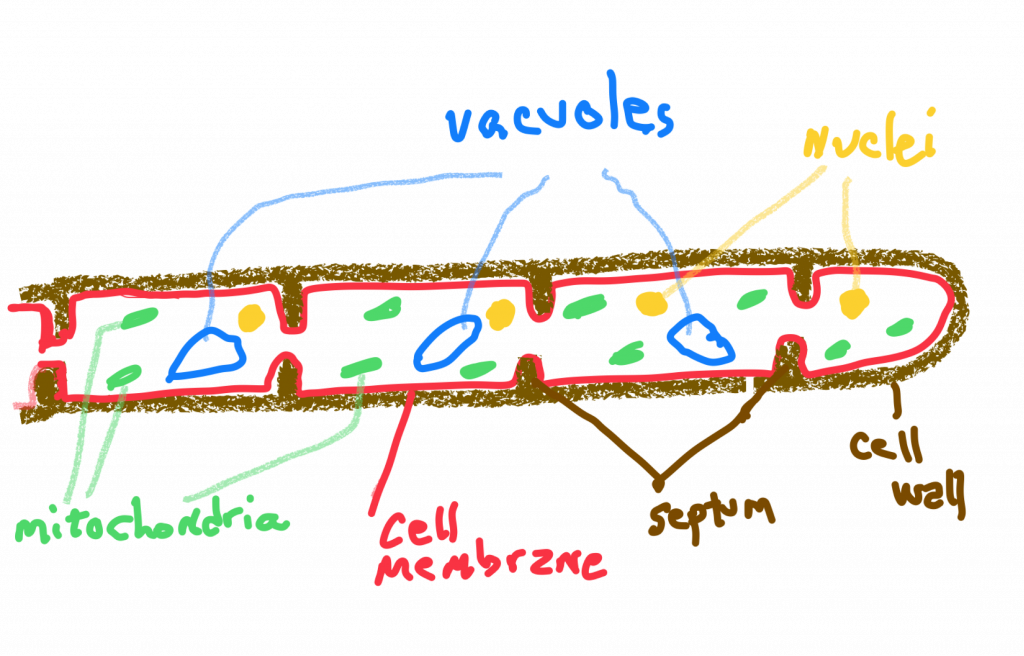
Two fungal groups are considered cellular because they do have cross-walls of sorts. These are called septa (singular septum) and they only partially seal off cells because they are perforated by pores that are large enough to allow many materials (ribosomes, mitochondria and sometimes nuclei) to move from one cell to another. Thus, the composition of these fungi is in between coenocytic and cellular. The cellular structure of specific groups of fungi are described below.
Chytrids
The fungal group considered most primitive (probably better described as the group that diverged at the earliest time from the rest of fungi) are the Chytridiomycota (chytrids). Chytrids are small aquatic organisms, both marine and freshwater, with some existing in the film of water surrounding soil particles. Most are microscopic, usually the size of a typical unicellular organism (less than 100 um). They may be truly unicellular (one nucleus) or coenocytic (multiple nuclei). Many produce forms that are roughly spherical. Several chytrids form what are called rhizoids, root-like extensions off of the main body that attach them to a source of nutrition, often pollen, the spores of other organisms, or a single cell of a living or dead multicellular organism. Besides attachment, the rhizoids also serve to increase surface area and allow for more effective digestion and absorption. A few chytrids form filaments (hyphae) that occasionally branch to form a very small mycelium. The group is distinguished from all other fungi by having flagellated, mobile, spores.
Coenocytic, filamentous fungi
Two additional fungal groups, the bread molds (Zygomycota) and the Glomeromycota, are substantially bigger than chytrids and produce a coenocytic hyphae and form mycelia. While both these groups lack specific cell types, some do form specific structures which one might term organs, except that they are parts of cells, not composed of cells. For many bread molds these structures, stolons, rhizoids and sporangiophores, are relatively large and can be seen with the naked eye. In the Glomeromycota the ‘organs’ are much smaller structures, but again are parts of cells, not cells. The Glomeromycota are the fungi that, together with plants, form endomycorrhizae, associations between plant roots and fungi. In endomycorrhizae, the fungus penetrates individual root cells (‘endo’ means inside) and inside these cells the fungus form two structures, tree-like arbuscules and spherical vesicles (endomycorrhizal fungi are sometimes called vesicular-arbuscular mycorrhizae or simply VA mycorrhizae). These structures are extensions off of the coenocytic hyphae. Arbuscules are structures with some similarities to the the rhizoids of chytrids. They serve no anchorage role but are significant in increasing the area of contact between the fungus and the plant, thereby allowing for greater material movement between them. Vesicles are spherical bodies that are thought to store materials for the fungus and which may develop into spores.
Septate fungi—Club and Sac Fungi
The two largest groups of fungi, club fungi (Basidiomycota) and sac fungi (Ascomycota) are not coenocytic. They produce hyphae that are septate, with cross walls to delineate individual cells. As the growing tip of a hypha extends, nuclear divisions (mitosis) are coordinated with the cellular divisions (cytokinesis) that produce new cross walls with a substantial pore. These cross walls are oriented perpendicular to the long axis of the hypha. As a result, new cells, each with a nucleus, are sequentially produced at the tip as it grows. The tip cell is also capable of occasionally dividing in a manner to cause the tip to bifurcate, to split in two, forming a branch. Branching sometimes also occurs away from the tip, the result of an outgrowth from a previously formed cell.
Under certain conditions the hyphae of Ascomycota and Basidiomycota may also form much more compact mycelia, usually associated with reproduction, and some of which approach in form structures that are more typical of multicellular organisms. These structures may range in organization from an amorphous mass of compact hyphae (aka a dense mycelium), termed a stroma, in which ‘fruiting bodies’ (spore producing structures) are formed (see tar-spot disease), to much more defined and determinate structure such as a typical mushroom with a stalk and cap. The stalk generally serves to enhance the dispersal of spores that are produced in the cap. Most of the organisms that produce mushrooms are club fungi (Basidiomycota) but a few are cup fungi (Ascomycota), and both groups produce fruiting structures that are not at all mushroom-like (e.g. bracket fungi). Both groups produce a wide variety of fruiting body forms, all developing as a result of pattern of growth of multiple hyphae. Although these structures are clearly organized and produce a consistent (determinate) three-dimensional final form, they generally show little evidence of tissues or specialized cell types, except those directly associated to sexual reproduction.
Another dense, compact hyphal structure produced by septate fungi is called a fungal cord or a rhizomorph. It consists of a multiple hyphae running parallel and glued to each other, forming a thread that may be several millimeters in diameter (cf. to a fungal hypha which typically is ten-times smaller in diameter than a human hair). Rhizomorphs are the result of repeated hyphal branching at very low angles to the orientation of the parent hyphae, consequently allowing the branch to stay closely associated and fused with the hypha that produced it. The result is a structure with little capacity to feed (or lose water) when compared to a diffuse mycelium, because of its reduced surface area and reduced penetration of its environment. But the rhizomorph is specialized for mobility and allows the fungus to traverse low-nutrient space or environmentally hostile (e.g., dry) space and potentially arrive in a more favorable location. When and if the rhizomorph arrives in a nutrient rich zone the growth form reverts to a diffuse mycelium suited for nutrient absorption. The extension of rhizomorphs requires transport of nutrients in order to feed the tips of hyphae (where growth is occurring) using nutrients that are being acquired at some distance from the tip. This transport function is served by specialized, large diameter hyphae, analogous to tubes found in brown algae and vascular plants. Another specialized cell type found in rhizomorphs, and located toward the outside of them, are thick walled ‘sheathing hyphae’ that have a structure again similar to some cells (fibers) found in vascular plants. These give the rhizomorph structural integrity and make it is less likely to be severed, something that is of much more consequence to a rhizomorph than to an individual hypha.
A few fungi produce structures called sclerotia, again a dense mass of hyphae, but sclerotia often develop into distinct forms and sometimes show specialization between a hard outer ‘rind’ and inner cells. The cells on the interior often have substantial food reserves and sclerotia are typically overwintering structures, structures that can become dormant. The stored food allows the sclerotium, which is sometimes dispersed, to survive the winter and resume growth when favorable conditions return.
Cellular Structure of Water Molds
The water molds, or Oomycota are similar in form to the bread molds (Zygomycota) with both groups being composed of coenocytic filaments, hyphae, that collectively form a mycelium but show no cellular specialization other than cells associated with reproduction. The group includes some important plant pathogens, including late blight of potato, sudden oak death syndrome and ‘damping off’ diseases. In spite of the name, they aren’t molds (fungi) and many are terrestrial not aquatic (although the representative of the group that is most commonly seen, at least if you have an aquarium, is a white fuzz occurring on dead fish). Water molds were once grouped with the fungi because of morphology but they are now considered to be in the heterokont grouping that also includes brown algae and diatoms.
Cellular Structure of Cellular Slime Molds
During the unicellular part of cellular slime mold’s existence there obviously is no cellular differentiation, but when aggregation occurs the cells acquire different fates and one might consider this to be reflective of complex multicellularity. In particular, and of evolutionary significance, only some cells of the aggregate ultimately end up producing reproductive cells. Other cells, e.g., the cells in the stalk of the spore producing structure, contribute to reproductive success by elevating the spore producing structure but gain no evolutionary advantage for ‘doing a good job’ since they are not necessarily represented in the next generation. This is in contrast to the more normal situation in multicellularity where all the cells of a multicellular organism are derivatives (and hence share a genetic composition) with the original ‘starter cell’, typically a zygote or a spore. Because of their unusual path to multicellularity, the determinators of cell fate in cellular slime molds is an area of active study. There are no obvious visual/structural differences between the cells of the slug or the fruiting structure but their positions are different, some are on the outside, some inside; some are near the front, some are behind. And position appears to be important in determining cell fate, including the position at the time of aggregation. Moreover, there is strong evidence that cell fate and ‘knowledge’ of position is a result of communication via chemical signals, one of the criteria that workers consider to be a defining aspect of complex multicellularity.
Cellular Structure of Algae
Algae are an artificial grouping of aquatic photosynthetic eukaryotic organisms whose classification remains in some flux. Many algae are unicellular or are colonial (‘simple multicellularity’) and do not show any cellular specialization except for cells associated with sex and reproduction. Most of these organisms are small: microscopic, or barely visible to the naked eye. But there are three groups of algae, colorfully named the red algae, brown algae and green algae, that include forms that are described as ‘macroalgae’. They are large (i.e., not microscopic, generally at least 0.1 m in extent) and with an organized structure, often consisting of organs: cylindric stems and branches, flattened blades, air sacs, root-like holdfasts and other features. Almost all of the brown algae and most of the red algae could be described this way. This ‘macro’ form is proportionately less common in the green algae where many members are smaller and where a considerable number of forms are unicellular or colonial (filaments). Using the more lenient definitions of multicellularity, most macroalgae would be considered multicellular. Using more restrictive definitions, in particular requiring intercellular connections and communication that allows for cooperation between cells, substantially reduces the number of macroalgae that are considered ‘truly multicellular’. Nonetheless, it appears that rigidly defined complex multicellularity has evolved independently at least two times in each of the three macroalgae groups (along with three times in the fungi, one time in animals and twice in bacteria).
Brown algae
This group used to be considered a phylum but now is considered as an entity further down the taxonomic scale (a class, or a family, or a level in between). While almost all brown algae are macroalgae, many lack cellular differentiation and an obvious tissue organization. The structure is basically filamentous, with the filaments branching and interacting with each other to form a distinct structure, similar to the way that certain fungi produce mushrooms out of hyphae. It appears that ‘true’ multicellularity, with the presence of cellular differentiation and tissues, has evolved twice in this group, once in a line that includes rockweed (Fucus) and once in a line that includes giant kelp (Laminaria). In both these groups there are distinct organs (holdfast, stipe, blade, air bladders) along with some cellular and tissue differentiation; in particular, there are cells specialized to allow for the long-distance movement of carbohydrates throughout the organism. These cells are analogous (not homologous!) with transport cells of vascular plants and have similar features: they are elongate cells with large diameters and with multiple trans-cellular cytoplasmic connections between adjacent cells. Another distinct cell type, also analogous with what is found in vascular plants, are cells with thickened cell walls that provide for structural integrity, allowing a large organism to hold together in spite of being pushed and pulled by ocean currents and/or waves.
Red Algae
The red algae group has roughly four times the number of species as the brown algae and is more diverse in terms of morphology. A substantial number are macroalgae with a definite organized structure. As was the case in fungi and some brown algae, their three-dimensional complexity is a consequence of the growth and interactions of multiple filaments. Tissues can be described in some forms, with the cylindrical portions (‘stems’) having distinct outer and inner layers and possessing cells distinguished by their size and pigmentation. Some red algae are crust forming (‘crustose’) and exist as a coating on the surface of various substrates. In many crustose forms there is a layered organization, with the filaments next to the substrate having different cells from those above. Some of the crustose red algae develop a layer of dividing cells near the top surface whose action allows the crust to thicken and also allows for the development of reproductive structures.
Green Algae
Of the three algal groups that include macroalgae, the green algae group is by far the largest (15,000 species), with many unicellular, simple filamentous and other colonial forms. Consequently, the macroalgae are a much smaller portion of the total species. Some of the macroalgae are siphonaceous, often composed of multinucleate filaments that interact to form a three-dimensional form. Most modern (cladistic) phylogenies divide the green algae into two groups, one of which, in addition to including (some) green algae, contains all of the organisms considered plants. Both groups exhibit a variety of forms, including some macroalgae with ‘complex’ multicellularity, showing three-dimensional growth, cellular connections and distinct cell types. Some of the green macroalgae (e.g. Caulerpa) are siphonaceous, producing a large complex form out of many large, multinucleate cells.
Further Reading and Viewing
- “Are mushrooms the new plastic?” by Eden Bayer. Interesting application of fungal structure to make organic “styrofoam” evocative design:
- “Amazing Science: Ten Craziest Things Cells Do” by Wallace Marshall. A video on remarkable cells.
Media Attributions
- Cells of Cork © Robert Hooke is licensed under a Public Domain license
- Diagram of Cells © Jrockley is licensed under a Public Domain license
- Ulothrix © Popular Science Monthly Volume 60 is licensed under a Public Domain license
- Oedogonium gametangia © Curtis Clark is licensed under a CC BY-SA (Attribution ShareAlike) license
- Anabena filaments © Cooke, M. C. (Mordecai Cubitt), b. 1825 is licensed under a Public Domain license