Chapter 19: Cellular Respiration
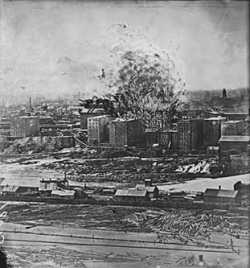
Although most bakers don’t realize it, they work with explosive material. Flour is highly flammable and under appropriate conditions flour dust can explode. On several occasions flour mills have exploded, perhaps the most famous being near Minneapolis in 1878 when a recently built mill, one that at the time was the largest in the world, was totally destroyed and 18 workers killed (Fig. 1). More recently, in 2008, a sugar mill in Georgia exploded, killing 14 and injuring 40 more. These examples demonstrate that there is energy present in carbohydrates, chemicals with the general formula of CH2O, i.e., a ‘hydrated’ carbon. Often the carbohydrates are polymers of six carbon sugars, molecules with a formula of C 6H12O6. Cane sugar, what exploded in the Georgia mill, is made up of two such ‘hexose’ (six-carbon) sugars, glucose and fructose, bound together. Starch, the main component of flour, is made up of long chains of glucose molecules bound together. Glucose, fructose and starch are all carbohydrates and like all molecules they ‘contain’ energy. If carbohydrates react with oxygen to form carbon dioxide and water, energy is released.
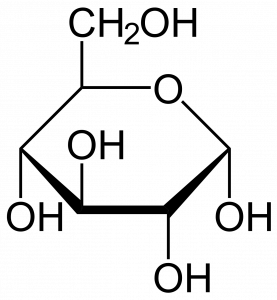
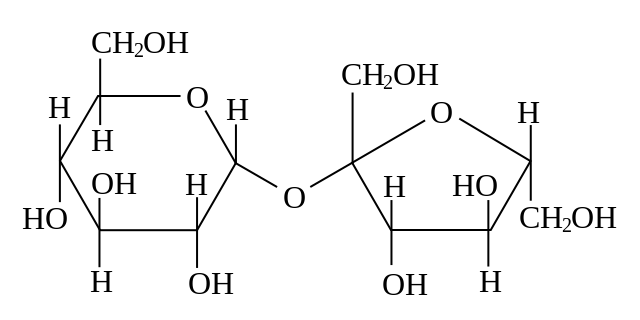
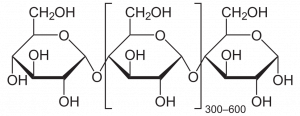
The energy of carbohydrates and its release when interacting with oxygen is central to the biology of most organisms. And understanding how the energy is obtained and utilized is significant not only because the energy released is essential for the functioning of organisms but also because it represents a unifying feature of all living things, every living organism carries out this process, or part of this process, or something similar to this process.
Organisms need energy for growth, maintenance, and for the performance of work such as the motion of the whole organism, e.g., swimming, or internal motion, e.g., pumping materials within the organism or moving materials within a cell, that are essential for the organism’s livelihood. Many, but certainly not all, of these energy-requiring processes ‘run’ on energy ‘supplied by’ adenosine triphosphate, ATP, and most of an organism’s supply of ATP is provided by cellular respiration, a process that synthesizes ATP while carrying out a chemical reaction that utilizes carbohydrates. Exactly how ATP participates in metabolism varies and its action is often not direct and obvious in the way that the energy of falling water allows a mill to ‘do work.’ The action of ATP often involves ‘coupling’ different chemical reactions (examples below) with the consequence being that the participation of ATP makes unlikely events more likely to happen and/or events that occur slowly more likely to proceed rapidly. ATP’s participation in cellular activities causes the molecule to lose one or two of its three phosphate groups, forming either adenosine diphosphate (ADP) or adenosine monophosphate (AMP). Obviously, the regeneration of ATP is significant to an organism’s functioning and for most organisms, this regeneration is the result of a group of reactions described as cellular respiration.
TOPICS
- Overview of cellular respiration
- Four parts of cellular respiration
- glycolysis: glucose to pyruvate
- pyruvate decarboxylation
- citric acid cycle (Kreb’s cycle)
- oxidative phosphorylation
- Mechanisms of ATP synthesis
- Summary
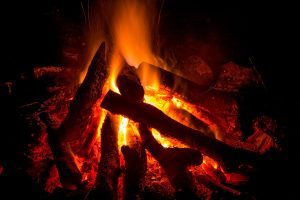
Overview of cellular respiration
Cellular respiration describes a set of chemical reactions that together convert carbohydrates and oxygen into carbon dioxide and water. Collectively, these reactions allow a cell to obtain chemical energy in the form of ATP from the same basic process that allows causes a flour mill to explode and allows campers to obtain heat and light (other forms of energy) when wood (which is largely carbohydrate) is burned in a campfire (Fig. 5). The chemical process of burning is an oxidation, or more properly a reduction/oxidation, a type of chemical reaction where an electron is transferred from one molecule to another. The molecule that loses electrons is said to be ‘oxidized’; the one that receives electrons is ‘reduced’. The process is generally ‘driven’ by the fact that some molecules/atoms have a higher affinity for electrons than others. In a thermodynamic sense, this is similar to the fact that rocks move downhill in response to gravity; one might say that ‘low spots’ (in a gravitational field) have a higher affinity for rocks than ‘high spots’. It takes energy to move rocks up in a gravitational field and (most of) the energy expended moving a rock up ‘ends up’ in the rock now in its new, higher position. If the rock then rolls down it ‘gives up’ energy and, after descending, the rock ends up with less energy than at the bottom than it had before. The first law of thermodynamics tells us that the energy is somewhere, where is it? One can state that the energy has been ‘released’ as rocks move downhill and also that this energy can be ‘captured’ in various ways, i.e., work can be done in the process (can you think of a way to capture the energy of a falling rock?). In a similar manner, electrons move ‘downhill’, from molecules that have less affinity for them to molecules that have a greater affinity for them, and as they move ‘downhill’ work can be done. Keeping track of electrons and the affinity of different compounds for electrons is sometimes challenging and we won’t pursue it in detail here, except to say that the task is made easier when what is transferred is an electron plus a proton, i.e., a hydrogen atom and in these cases the oxidation/reduction is easy to trace by seeing what loses hydrogens and what gains hydrogens.
The summary equations for cellular respiration, in words and formula, are:
- carbohydrate plus oxygen forms carbon dioxide plus water
- specifically, glucose plus oxygen forms carbon dioxide plus water
- C6 H12 O6 + 6 O2 —— > 6 CO2 + 6 H2 O
In cellular respiration what is oxidized are the carbons in a carbohydrate molecule of the general formula CnH2nOn and what is reduced is O2. The carbons of the carbohydrate have lost hydrogens while forming carbon dioxide (CO2). The oxygen has gained hydrogens while forming water (H2O). It is important to realize that the carbohydrate does NOT react with oxygen (although it does if a log is burned in a fire) ; the equation merely summarizes a group of reactions occurring simultaneously and have the net effect of converting carbohydrates and oxygen to carbon dioxide and water.
We can split these reactions into four basic parts:
-
Glycolysis
A process that converts a glucose (six carbon sugar with six carbons, 12 hydrogens and six oxygens) into two pyruvic acid molecules, each with three carbon molecules and with the formula C3H4O3 (Fig. 6). Note that the carbons of the carbohydrate have been oxidized (lost hydrogens) as pyruvic acid has been formed. What has been reduced is a metabolite called NAD+ which has been reduced to NADH. Because the NAD+ has a higher affinity for hydrogens than the carbon in a carbohydrate, this group of reactions is ‘downhill’. Also occurring in glycolysis is the synthesis of some ATP from ADP and phosphate ion (iP). Thus, some energy present in the hexose is now present in the forms of NADH and ATP (and some has been released heat and some is present in the pyruvic acid molecules).
Fig. 6 The reactions of glycolysis, starting with glucose (C6H12O6) and ending with the production of two molecules of pyruvic acid (C3H4O3). Note that fructose 1, 6 bisphosphate is split into two three carbon molecules, GAP and DHAP, and the DHAP is converted into GAP. -
Pyruvic acid decarboxylation
Each of the two pyruvic acid molecules is ‘oxidatively decarboxylated’, removing a carbon as a carbon dioxide and producing a two carbon (‘acetyl’) fragment attached to the metabolite coenzyme A. The lost carbon has been oxidized as NAD+ is reduced to NADH. Because NAD+ has a higher affinity for electrons than the carbon in the pyruvic acid this reaction is ‘downhill’.
-
Krebs cycle
The remaining two carbons derived from each pyruvic acid are added to a four-carbon compound making a six carbon compound that is then oxidatively decarboxylated twice and then goes through a series of oxidative steps to regenerate the original four carbon compound that can receive another two-carbon unit. This is what is known as both the Kreb’s cycle or the citric acid cycle.
The net effect of steps 1-3 is the total oxidation of the carbohydrate to carbon dioxide, accompanied by the reduction of a number of NAD+ molecules to NADH and also the reduction of a similar molecule, FAD, to FADH2. Some ATP has been synthesized in both glycolysis and the citric acid cycle, but the majority of the ATP generated in cellular respiration comes from step 4 below where the ‘reducing power’ of NADH and FADH2 is utilized to ‘power’ an elaborate mechanism that ‘utilizes’ energy from the reduced molecules to accumulate protons and create a charge and concentration gradient across a membrane. This gradient is then used to synthesize ATP.
-
Oxidative phosphorylation (electron transport chain)
Like the citric acid cycle, oxidative phosphorylation occurs in a mitochondrion, an organelle with two membranes with two aqueous spaces: in between the two membranes and inside the inner membrane (Fig. 7). Oxidative phosphorylation transfers electrons, donated by NADH and FADH2, through a series of membrane bound carrier molecules located in the inner membrane, ultimately delivering them to oxygen. Oxygen simultaneously picks up protons and form s a water molecule, H2O. Oxygen is essential to the process because it is oxygen’s affinity for electrons that drives the electron movement. To again use a gravitational analogy, oxygen is a ‘low point’ to which electrons move. If there isn’t a low point there would be no movement. The movement of electrons through a membrane operates an ‘electrogenic pump’: it causes protons (H+) to accumulate in the space between the two membranes, creating an electrochemical gradient, a charge and concentration difference, across the membrane. In an energetic sense, the pumping is ‘uphill’ and it is made possible by a coupling to the ‘downhill’ movement of electrons to molecules with higher affinity for them. The proton gradient thus created is a source of energy that can be utilized to synthesize ATP from ADP and iP. In oxidative phosphorylation there are two things moving ‘downhill’ in an energetic sense: (1) electrons move from NADH ‘downhill’ to oxygen because it has a higher affinity for them, (2) protons that have been ‘pumped’ uphill then move ‘downhill’ across a membrane, from a place where they are in high concentration to a place where they are in low concentration. If one ‘follows’ the energy it goes from glucose to the reducing power of NADH/FADH2, to a proton electrochemical gradient, to ATP. Besides producing ATP, another very important consequence of the process of oxidative phosphorylation is the regeneration NAD+ and FAD, compounds that are needed in glycolysis and the citric acid cycle. These processes cannot proceed unless NAD+ and FAD are available (this point will be discussed further in Chapter 21 on metabolic diversity).
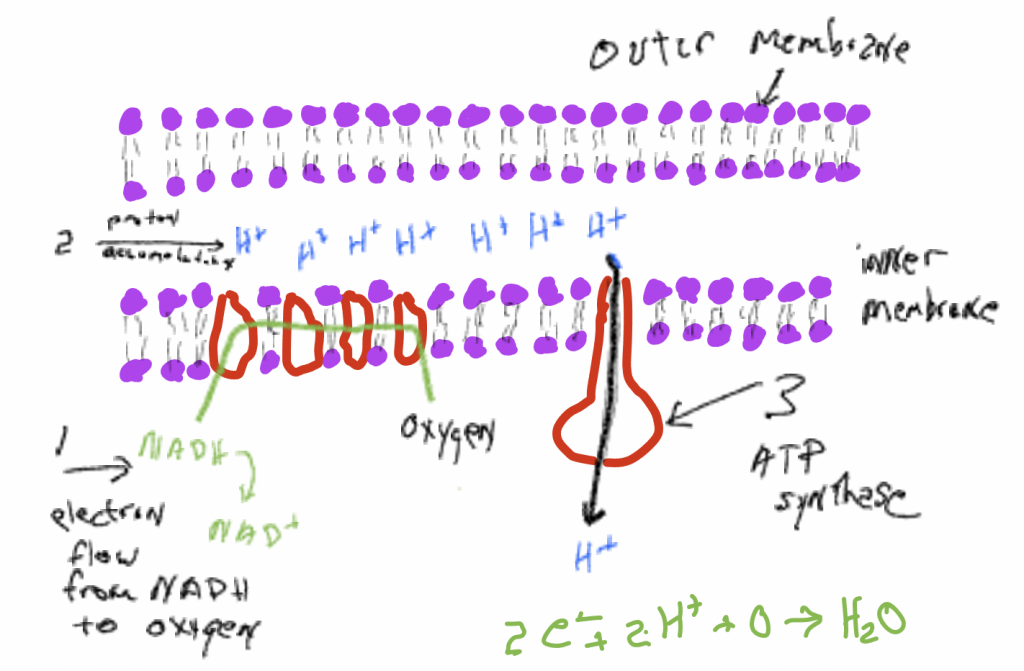
Mechanisms of ATP synthesis
During cellular respiration ATP is formed in two very different ways, both of which involve energy transfers and the concept of ‘coupling’, in these cases the coupling of ATP synthesis to other reactions that ‘provide energy’. Examining these reactions is not only important to the energy relations of a cell, but they also provide examples of ‘coupling’.
ATP is formed when a phosphate group (PO3–) is added to ADP, an ‘uphill’ reaction with ATP having more energy than ADP plus phosphate and under most circumstances, this reaction is very unlikely. Most of the ATP formed by eukaryotic living things occurs in organelles called mitochondria where the electron transport chain discussed above results in a high concentration of protons on the outside of a membrane (remember that membranes are generally impermeable to charged items like protons). Embedded in the membrane, with openings to both sides, is a large enzyme, a polymer of amino acids, that has a very specific and complicated three-dimensional structure, a structure that is a consequence of the sequence of amino acids in the polymer. This enzyme binds ADP and phosphate and also has a path, a channel, through which protons can flow through the protein (and also through the membrane) from high concentration to low. The movement of protons through the protein causes the enzyme with attached ADP and phosphate to be bent in a way that makes it much more likely that phosphate binds to ADP, thereby forming ATP. Note that the charge and concentration difference of protons across the membrane represents a ‘source of energy’ that can be used to do things, in this case, bend the molecule and synthesize ATP. The energy from the proton gradient makes an unlikely reaction, ATP synthesis, much more likely. Stated another way, ATP synthesis is coupled to protons moving from high concentration to low.
A second way to synthesize ATP from ADP and phosphate is seen in glycolysis. Instead of directly transferring a phosphate group to ADP it is first added to GAP (Figure 1). This reaction by itself is unlikely (uphill) but it can be made more likely if the GAP is simultaneously oxidized while NAD+ is being reduced, a reaction that is favored (downhill) because NAD+ has a higher affinity for electrons than GAP. The combined reaction results in the conversion of GAP to 1,3 bPG, with the GAP being simultaneously oxidized and phosphorylated. And while the attachment of a phosphate group directly to ADP is ‘uphill’ (unlikely), the transfer of a phosphate group from the 1,3 bPG to ADP is ‘downhill’ (likely), thus there is coupling between oxidation of GAP and ATP synthesis. A similar mechanism operates where ATP is formed in the citric acid cycle. Coupling is an important aspect of both ATP synthesis and hydrolysis and represents ways that energetically unfavorable (uphill) reactions can be made more likely by coupling them somehow (and there are multiple ways) to favorable (downhill) reactions.
Summary
Thus the net effect of cellular respiration is the complete oxidation of carbohydrates to form carbon dioxide and water. In the process, ATP is synthesized from ADP and phosphate. Although the process as described above ‘starts’ with glucose, a number of other molecules can provide six carbon sugars to be utilized in glycolysis, e.g., starch (a polymer of glucose), sucrose (a disaccharide containing glucose and fructose), galactose (a six carbon sugar), lactose (a disaccharide made of glucose plus galactose), mannitol (a six-carbon sugar alcohol). In plants, sucrose and starch are the most important sources of substrates for glycolysis. Besides hexose/hexose polymers, other materials can be ‘burned’ in cellular respiration, including fats and the carbohydrate portion of the amino acids of proteins. These ‘food sources’ enter the metabolic pathways of cellular respiration in several different places.
Although cellular respiration is generally thought of as being a degradative process (catabolism), it can also be synthetic (anabolism—the making of biomolecules). When this happens the material entering the cellular respiration exits the process before being completely oxidized, thereby providing metabolites that are used to construct biomolecules. For example, intermediates of cellular respiration can be used to synthesize fats and amino acids. When this happens less (or no) ATP energy is obtained because less of the process of cellular respiration is occurring.
Further Reading and Viewing
Ion the spring, skunk cabbage flowers generate heat by decoupling proton flow from ATP synthesis (as do some animals when they emerge from hibernation). Energy that might have been “used” to make ATP is released as heat. Learn more about this interesting plant in Plants are Cool, Too! Episode 3 on YouTube and in the article “Living together and living apart: the sexual lives of bryophytes” by David Haig published by the Royal Society.
Media Attributions
- Washburn A Mill Explosion © Jacoby is licensed under a Public Domain license
- Glucose © NEUROtiker is licensed under a Public Domain license
- Sucrose
- Starch © NEUROtiker is licensed under a Public Domain license
- Campfire © Alasdair is licensed under a CC BY (Attribution) license
- Reactions of glycolysis © http://www.wikiwand.com/als/Stoffwechsel is licensed under a CC BY (Attribution) license